- 1Department of General Surgery, Qilu Hospital, Shandong University, Jinan, Shandong, China
- 2Department of General Surgery, Qilu Hospital, Shandong University, Qingdao, Shandong, China
Cholangiocarcinoma (CCA) is a highly aggressive malignant tumor that originates from the biliary system. With restricted treatment options at hand, the challenging aspect of early CCA diagnosis leads to a bleak prognosis. Besides the intrinsic characteristics of tumor cells, the generation and progression of CCA are profoundly influenced by the tumor microenvironment, which engages in intricate interactions with cholangiocarcinoma cells. Of notable significance is the role of extracellular vesicles as key carriers in enabling communication between cancer cells and the tumor microenvironment. This review aims to provide a comprehensive overview of current research examining the interplay between extracellular vesicles and the tumor microenvironment in the context of CCA. Specifically, we will emphasize the significant contributions of extracellular vesicles in molding the CCA microenvironment and explore their potential applications in the diagnosis, prognosis assessment, and therapeutic strategies for this aggressive malignancy.
1 Introduction
Cholangiocarcinoma (CCA) is the predominant form of primary malignant tumor originating from the biliary system (Blechacz et al., 2011). It can be categorized into three subtypes based on the anatomical site involved: intrahepatic cholangiocarcinoma (iCCA), perihilar cholangiocarcinoma (pCCA), and distal cholangiocarcinoma (dCCA). The worldwide occurrence of CCA has exhibited a consistent rise over the past four decades (Banales et al., 2020). CCA is highly invasive, and its clinical symptoms in the early stages are often subtle, leading to diagnostic challenges. Most patients receive a diagnosis when the disease has already progressed to an advanced stage. Surgical resection, often seen as the sole potentially curative treatment, is a viable option for around 20%–30% of patients. When combined with adjuvant capecitabine, resection can lead to a reported median survival of 53 months. Regrettably, for the remaining 70%–80% of patients with locally unresectable or distant metastatic disease, survival tends to be restricted to approximately 1 year (Moris et al., 2023).
Cholangiocarcinoma (CCA) is a desmoplastic hyperplastic tumor characterized by a unique tumor microenvironment (TME) (Wang and Ilyas, 2021). Beyond the influence of tumor cell epigenetics, tumor formation and advancement are primarily governed by the TME (Baghban et al., 2020). Reprogramming of tumor initiation, growth, invasion, metastasis, and the response to therapy heavily relies on the crucial role played by the TME (Jin and Jin, 2020). The interplay between tumor cells and the TME is a bidirectional, ever-changing process, involving both cell-cell and cell-free contact through various signaling molecules and extracellular vesicles (Figure 1). These secreted soluble molecules, cytokines, and extracellular vesicles are responsible for the transfer of genetic information horizontally among cells, facilitating cell-to-cell communication (Baghban et al., 2020). Among these, extracellular vesicles stand out as crucial carriers in mediating intercellular communication between tumor cells and the TME (Lemoinne et al., 2014).
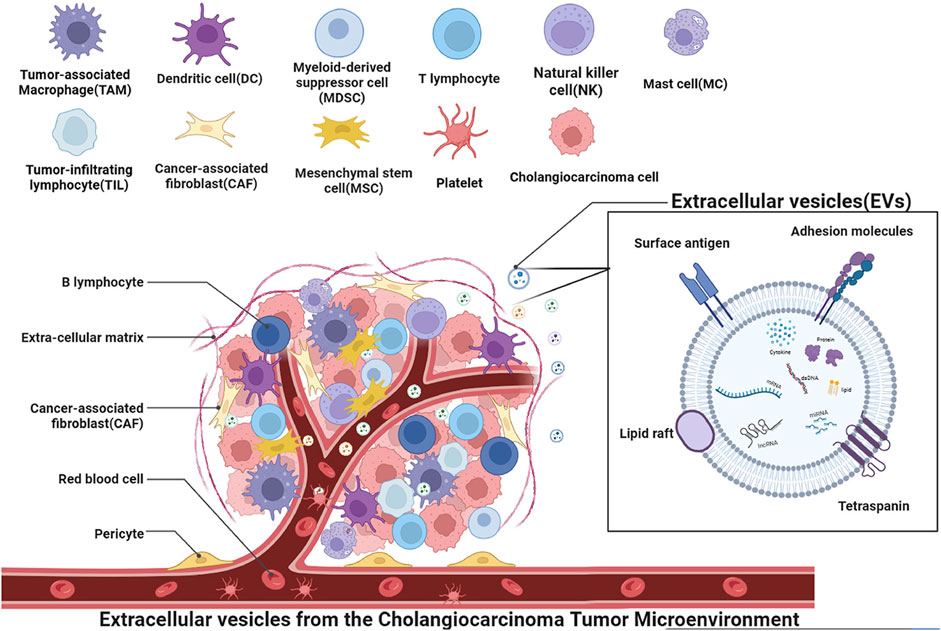
FIGURE 1. Schematic depiction of the role of extracellular vesicles in the context of cholangiocarcinoma microenvironment cells. The microenvironment is comprised of cancer cells, mesenchymal stem cells, cancer-associated fibroblasts, immune cells such as tumor-associated macrophages (TAM), mast cells (MC), natural killer cells (NK), tumor-infiltrating lymphocytes (TIL), vascular endothelial cells, and platelets associated with angiogenesis. Various cells interact through vesicles to form the cholangiocarcinoma tumor microenvironment, promoting tumor invasion, regulating tumor growth, promoting angiogenesis, and performing other functions. Extracellular vesicles, as a mode of transportation, can transfer various substances such as mRNA, lncRNA, miRNA, dsDNA, signaling proteins, lipids, cytokines, and other extracellular vesicles to target cells. These substances can regulate key processes in tumor progression.
Extracellular vesicles (EVs) are lipid bilayer spheres that encapsulate signaling proteins, lipids, nucleic acids (DNA and RNA), and metabolites, and they are released by diverse cell types into the extracellular media of various biological fluids, including serum, urine, bile, and saliva (Kahlert et al., 2014; Melo et al., 2015). Extracellular vesicles have the capacity to be conveyed to nearby or remote cells via a range of mechanisms, including direct interaction with the cellular membrane, fusion, or internalization (Colombo et al., 2014). These EVs carry signals to recipient cells and participate in intercellular communication in both physiological and pathological conditions. EVs are categorized based on their biogenesis into exosomes, microvesicles (MVs) or microparticles, and apoptotic bodies. Exosomes, which are produced within the multivesicular endosomes (MVEs) of cells, exhibit a spherical morphology and a diameter ranging from 40 to 150 nm. MVEs, generated during the maturation process of early endosomes, give rise to intraluminal vesicles (ILVs) through the invagination of the MVE membrane. Upon fusion of MVEs with the cell’s plasma membrane, ILVs are released into the extracellular media as exosomes. In contrast, MVs or microparticles originate from the direct budding of the cell’s plasma membrane and display heterogeneous sizes (ranging from 40 to 1,000 nm) and morphologies (Raposo and Stoorvogel, 2013). Apoptotic bodies, on the other hand, are vesicles produced by cells undergoing apoptosis, characterized by diverse sizes (∼40 to 2000–5,000 nm) and morphologies (Akers et al., 2013). EVs possess the remarkable ability to be transported to neighboring or distant cells through various mechanisms, including direct interaction with the cellular membrane, fusion, or internalization. Among these vesicles, exosomes have garnered greater research attention in this review due to their heightened significance. Bioactive substances carried by EVs exert regulatory control over crucial processes in tumor progression, such as inflammation promotion (Haga et al., 2015), reconfiguration of the cellular-matrix interface (Mu et al., 2013), angiogenesis (Bouvy et al., 2014), chemotherapeutic drug resistance (Chen et al., 2014; Shao et al., 2015), as well as suppression of anti-tumor immune responses (Chalmin et al., 2010). This paper delves into the intricate interactions among different components within the cholangiocarcinoma microenvironment, with a particular emphasis on elucidating the contribution of EVs.
2 The role of contents carried by extracellular vesicles in tumor microenvironment
Extracellular vesicles (EVs) serve as vital mediators of intercellular communication within TME (Lindoso et al., 2017), and they exhibit a remarkable degree of heterogeneity and dynamism, with their content, size, and membrane composition being contingent upon factors Including factors like the cellular source, its current state, and the surrounding environmental context (Yáñez-Mó et al., 2015). In this context, EVs assume a central role in coordinating the exchange of intercellular information, as they fuse with target cells to deliver a spectrum of contents (Figure 2), including proteins, lipids, mRNA, microRNAs, long non-coding RNAs (lncRNA), double-stranded DNA (dsDNA), and various other bioactive molecules (Raposo and Stoorvogel, 2013).
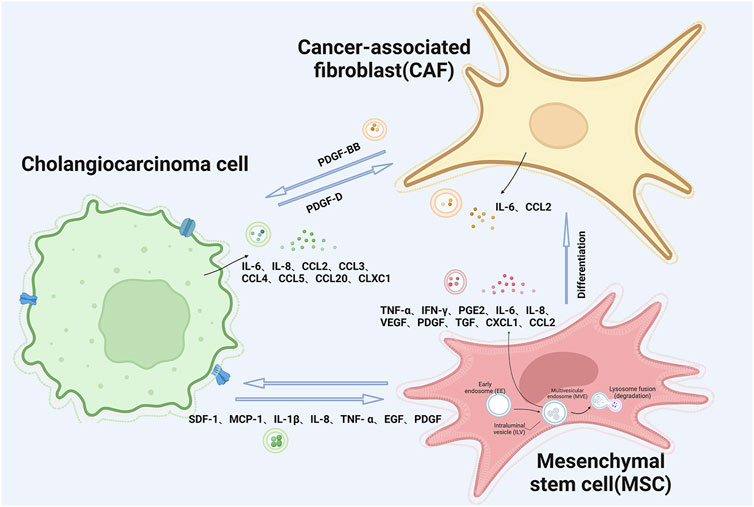
FIGURE 2. Schematic illustration of extracellular vesicle interactions between cholangiocarcinoma tumor cells and mesenchymal stem cells and cancer-associated fibroblasts.
In CCA and its TME, EVs secreted by CCA cells stimulate cholangiocyte proliferation and promote their invasive behavior, and these events are related to the enrichment of oncoproteins in EVs. Proteins with varying expression patterns that are implicated in the progression of CCA encompass epidermal growth factor receptor (EGFR), Mucin-1, and Integrin β 4 (ITGB4), epithelial cell adhesion molecule (EPCAM) (Arbelaiz et al., 2017), Galactose Lectin 3 binding protein (LG3BP), Prostaglandin F2 receptor negative regulator (PTGFRN) and 4F2 cell surface atigen heavy chain (4F2hc) (Dutta et al., 2015), etc. EGFR contributes to tumor cell dedifferentiation and invasiveness, serving as an unfavorable prognostic indicator. Meanwhile, the upregulation of Mucin-1 and EPCAM in CCA is linked to an unfavorable prognosis among CCA patients (Clapéron et al., 2014). ITGB4 has been identified as an EV-associated integrin that plays a pivotal role in dictating the future metastatic site, thereby contributing to the selective organ-specific alignment of tumor cells (Hoshino et al., 2015). The serum exosomes of CCA are enriched in several proteins, such as aminopeptidase N (APN), pantothenate (VNN1), and polyimmunoglobulin receptor (PIGR) (Arbelaiz et al., 2017). Compared with primary sclerosing cholangitis (PSC) samples, fibrinogen gamma chain (FGG), α-1-acidic glycoprotein 1 (A1AG1), and S100A8 were stable in the EV of CCA samples (Sirica et al., 2019). These investigations suggest that the proteins found in exosomes have the potential to be regarded as early diagnostic indicators for CCA. Proteins transported by EVs as carriers in the tumor microenvironment of CCA are summarized in Table 1. Exosomes are capable of transporting a variety of bioactive lipids, including sphingomyelin, cholesterol, lysophosphatidylcholine, arachidonic acid, various fatty acids, prostaglandins, and leukotrienes, to diverse cellular destinations (Record et al., 2014). Research has shown that vesicle-bound lysophosphatidylcholine (Table 2) supports DC maturation and lymphocyte chemotaxis via G protein-coupled receptors (Subra et al., 2007). Additionally, vesicle-bound prostaglandins (Table 2) activate intracellular signaling pathways, such as PGE2, promoting immunosuppression for tumor development (Subra et al., 2010). Prior research has provided evidence that sphingomyelin (Table 2) primarily mediates the angiogenic activity of tumor-derived EVs in vitro and in vivo (Kim et al., 2002). However, while lipomics and exploring the complete lipid profile of EVs are emerging research areas, current lipid separation and analysis technologies limit the description of only a few lipid groups.
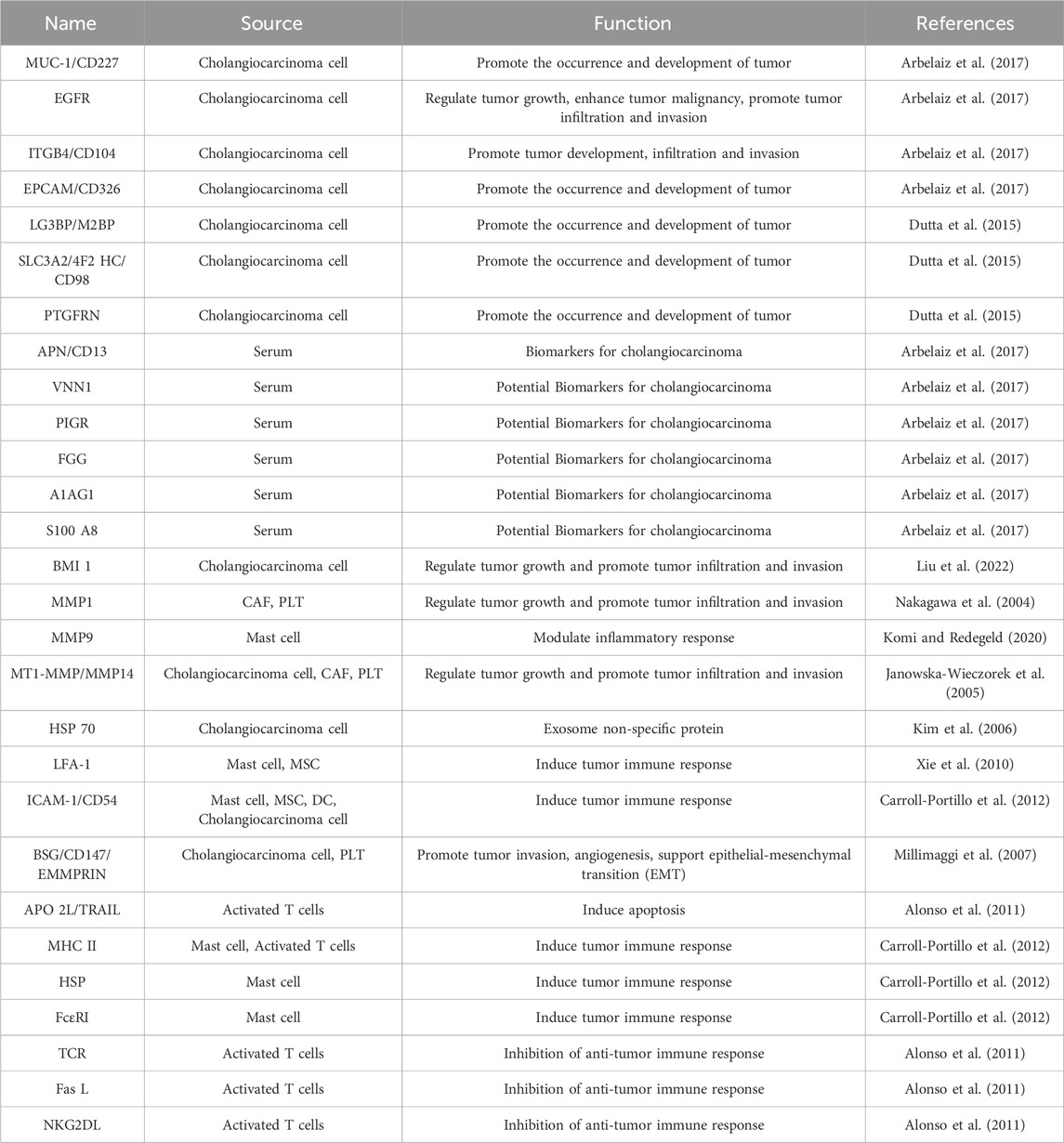
TABLE 1. Proteins of extracellular vesicles specifically expressed in the tumor microenvironment of cholangiocarcinoma.
The extracellular vesicles contain intact mRNA (Zuo et al., 2020), mRNA fragments (Lu et al., 2017), long non-coding RNAs(Liechty et al., 2000), microRNA (miRNA) (De Bruyn et al., 2011), etc. In certain cellular contexts, miRNA can be transported to neighboring cells via EVs, consequently modulating the gene expression and phenotypic traits of the receiving cells. RNAs transported by EVs in the CCA tumor microenvironment mentioned in this article is summarized in Table 3.
EVs secreted by cancer cells are reported to contain a higher quantity of DNA fragments compared to those from normal cells. Notably, tumor extracellular vesicles harbor DNA reflecting the tumor’s genetic state, including the amplification of oncogenic gene c-myc (Balaj et al., 2011). These EVs are capable of transferring DNA to target cells, where the presence of double-stranded DNA (dsDNA) representing genomic DNA has been detected (Waldenström et al., 2012). Furthermore, detecting mutations within exosomal DNA has displayed substantial promise as a circulating diagnostic biomarker for cancer within clinical environments (Thakur et al., 2014).
3 Extracellular vesicles influence and shape tumor microenvironment by secreting cytokines
Interactions among the diverse cells within the tumor microenvironment are primarily mediated by soluble molecules. The tumor microenvironment (TME) can be likened to a persistent site of inflammation, characterized by a plethora of infiltrating and endogenous cells responsible for the synthesis and secretion of cytokines, chemokines, and growth factors, including but not limited to TNF-α, MMP-9, IL-6, and VEGF. These molecules possess the capability to orchestrate and modulate inflammatory responses (Komi and Redegeld, 2020). Recent research indicates that EVs from CCA cells trigger the release of pro-inflammatory cytokines and chemokines, such as IL-6, CXCL1, and CCL2, from cells in the TME (Figure 2). In reaction to this sequence of events, it promotes the proliferation of CCA cells via activation of the IL-6/STAT3 signaling pathway (Haga et al., 2015). The release of IL-6 by mesenchymal stem cells (MSCs) leads to an upsurge in the production of endothelin-1 by tumor cells, which triggers the activation of Akt and ERK pathways in endothelial cells, thereby amplifying their recruitment to the tumor site and bolstering the angiogenic processes (Huang et al., 2013).
3.1 IL-6 as the significant cytokine in the TME of CCA
IL-6 assumes a pivotal role in mediating communication between tumor epithelial cells and the established TME. Its expression in response to tumor cell-derived EVs is strongly associated with tumor growth. IL-6 holds a particularly crucial position in the initial phases of malignancy development within the biliary epithelium. In instances of CCA associated with oncogenic risk factors like trematode infection, cholestasis, primary sclerosing cholangitis (PSC), or biliary atresia, the underlying inflammatory condition of the liver can stimulate heightened IL-6 secretion (Chaiyadet et al., 2015; Liu et al., 2015). Other studies have demonstrated that enhanced telomerase activity, triggered by IL-6 incentive, can inhibit cellular senescence in malignant cholangiocytes, thereby promoting CCA growth (Yamada et al., 2015). IL-6 in promoting cholangiocarcinoma growth has been acknowledged (Braconi et al., 2010).
3.2 Chemokines represented by CXCL1 and CCL2
On the other hand, factors such as CXCL1 do not appear to influence cell proliferation significantly. There is speculation that these factors may exert additional effects that have the potential to influence tumor progression or metastasis. CXCL1 is involved in processes such as angiogenesis, inflammation, tissue repair, and oncogenesis (Haghnegahdar et al., 2000). Recent research has revealed that CXCL1, a CXCR2 ligand, significantly reduces the growth and mobility potential of CCA cells. Studies indicate a negative correlation exists between the expression of CXCL1 and the occurrence of distant metastasis, suggesting CXCL1’s role as a CCA progression suppressor. Additionally, CXCL1-CXCR2 signaling induces cancer cell senescence, triggering anti-proliferative responses and apoptosis. These mechanisms contribute to CXCL1’s tumor-suppressive effect, highlighting its potential in CCA progression. Overall, The CXCL1-CXCR2 axis could potentially exert a tumor-inhibitory function in the progression of CCA (Yamamoto et al., 2022). Conversely, monocyte chemoattractant protein-1 (MCP1/CCL2), a known target chemokine of FAK, serves as a robust catalyst for the invasion and migration of cancer cells (Dwyer et al., 2021). Currently, there is limited research on chemokines’ role in bile duct cancer, mainly due to the prominence of CCL2 in this process. Cytokines and growth factors transported by EVs in the TME of CCA mentioned in this article is summarized in Tables 4, 5.
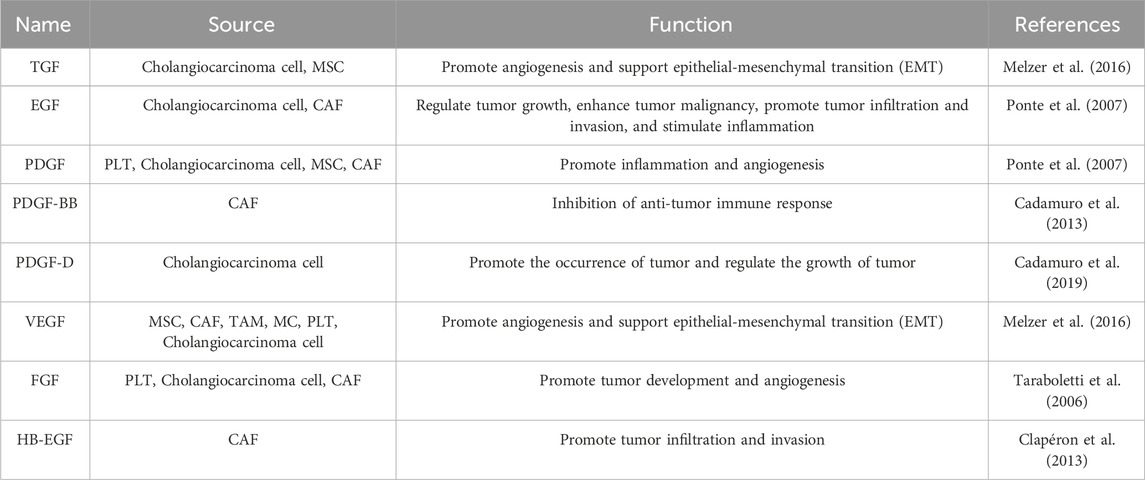
TABLE 5. Growth factors of extracellular vesicles specifically expressed in the tumor microenvironment.
3.3 Multiple cells secrete and induce growth factors
In the TME of CCA, multiple cells, including tumor-associated macrophages (TAMs), cancer-associated fibroblasts (CAFs), and cancer cells, have the capability to secrete and stimulate the production of growth factors. Fibroblast activation in CCA is primarily driven by factors such as transforming growth factor-β (TGF-β), fibroblast growth factor (FGF), and platelet-derived growth factor (PDGF), which are secreted by TAMs and CCA cells. When activated, CAFs secrete various growth factors, including PDGF, heparin-binding EGF-like growth factor (HB-EGF), and vascular endothelial growth factor (VEGF), all of which play crucial roles in promoting cancer progression. PDGFs, particularly PDGF-D produced by CCA cells, function in a paracrine manner and exhibit proto-oncogenic effects. PDGF-D is instrumental in the recruitment and activation of CAFs by binding to PDGFRβ on their surfaces (Cadamuro et al., 2019). Growth factors transported and released by EVs are implicated in the advancement of CCA by promoting tumor growth, local invasion, migration, as well as new blood and lymph vessel formation, and the metastatic spread of tumor cells.
4 Extracellular vesicles and mesenchymal stem cells
MSCs within the TME exhibit the capacity to transform into various cell types and engage with cancer cells, thereby advancing tumor progression and metastasis. Multiple studies have elucidated the pro-tumorigenic influences of MSCs, foremost among them being (Ⅰ) the instigation of angiogenesis (Huang et al., 2013), (Ⅱ) drug resistance (Balakrishnan et al., 2010), (Ⅲ) immune response evasion (Poggi and Giuliani, 2016), (Ⅳ) stimulating EMT (Mele et al., 2014), and (Ⅴ) promoting metastasis (McAndrews et al., 2015). The interplay between MSCs and tumor cells initiates distinct reactions in both cell types, facilitated through direct and indirect connections. Indirect interactions are typified by the release of various molecules responsible for dampening immune responses, including TNF-α, IFN-γ, PGE2, and IL-6, IL-8, VEGF, PDGF, and TGF related to the process of angiogenesis (Melzer et al., 2016), as mentioned above, these cytokines, chemokines are delivered by tumor microenvironment EVs. Notably, MSC-derived extracellular vesicles (EVs) have also been found to facilitate immunosuppression, promote M2 macrophage polarization, and induce Treg cell formation (Zhang et al., 2014).
TME produce inflammatory reactions to recruit MSCs (Spaeth et al., 2008). Tumors secrete a variety of chemokines and cytokines, which have been demonstrated to engage with receptors on MSCs, including stromal cell-derived factor-1(SDF-1), epidermal growth factor (EGF), PDGF, monocyte chemotactic protein-1 (MCP-1), IL-8, and IL-1β, and TNF- α. These has been proven to stimulate the orientation of MSCs toward the tumor niche (Ponte et al., 2007). MSCs are recruited in reaction to inflammation with a specific attraction or affinity (Kerkelä et al., 2013). After implantation into the inflammation site, MSCs can engage with neighboring cells through either direct physical contact or through paracrine signaling effects (Zanotti et al., 2013). In the presence of chronic damage or inflammation, MSCs are readily recruited into the biliary tract, fostering bile duct cancer development (Braconi and Patel, 2010). This understanding paves the way to formulate approaches designed to inhibit the onset and advancement of CCA.
CCA induces MSCs via EVs, impacting the TME and promoting tumor growth. CCA releases EVs, like exosomes, taken up by MSCs, leading to increased mRNA transcription and secretion of CXCL-1, CCL2, and IL-6. Exposure to these EVs also triggers α-smooth muscle actin mRNA expression, selectively boosting myofibroblast biomarker α-smooth muscle actin and FAP in MSCs, enhancing their migratory abilities and possibly forming a cell-matrix transition. MSC-conditioned medium exposed to tumor-derived EVs activates STAT-3 phosphorylation, boosting tumor cell proliferation (Haga et al., 2015). Tumor cell-derived EVs elevate MSC fibroblast-like activity, promoting tumor matrix production through fibroblast differentiation. These EVs specifically influence the secretion of soluble substances, including IL-6, from MSCs, impacting tumor cell proliferation. These alterations in the release of cytokines and the differentiation of fibroblasts play a role in the promotion of tumor cell proliferation and the formation of the CCA stroma (Yang et al., 2015). MSCs are central to promoting cholangiocarcinoma initiation and progression by interacting with diverse TME cells, offering novel insights for both treatment and prevention.
5 Extracellular vesicles and cancer-associated fibroblasts
Cancer-associated fibroblasts (CAFs) are integral to shaping the tumor microenvironment, fueling tumor invasion, proliferation, and metastasis, thus fostering malignant tumor progression (De Wever et al., 2014). Recent insights emphasize the function of exosomes in facilitating communication between CAFs and cancer cells (Yang et al., 2017). CAFs secrete growth factors, chemokines, pro-inflammatory mediators, matrix metalloproteinases (MMPs), and extracellular matrix (ECM) constituents, orchestrating the expansion of tumors, the promotion of angiogenesis, and the attraction of bone marrow-derived cells to primary tumor sites, ultimately facilitating metastasis (Fullár et al., 2012; Deng et al., 2017). Inappropriate or abnormal activation of signaling pathways, such as IL-6/STAT3, FGF-2/FGFR1, NF-κB, and TGF-β1/SMAD, distinguishes CAFs from normal fibroblasts (NFs). CAFs release specific exosomes, which are taken up by cancer cells, facilitating the transfer of various bioactive substances. Reciprocally, cancer cell-derived EVs promote CAFs conversion. This complex interaction is a contributing factor to the advancement and development of the tumor.
CAFs are the CCA matrix primary components, crucial in mediating its growth and progression. In CCA, their abundance correlates with tumor expansion and poorer survival (Banales et al., 2020). CAFs, through the secretion of immunomodulatory factors, exert significant control over the TME. They regulate the innate immune response by promoting the M2 polarization of macrophages and inhibiting the activation of natural killer (NK) cells. Furthermore, CAFs stimulate the generation of regulatory T cells and T helper 2 cells while hindering the activity of dendritic cells and cytotoxic T cells, influencing adaptive immunity dynamics (Banales et al., 2016). In iCCA, CAFs exhibit heightened expression of IL-6, and the IL-6/IL-6R axis is heightened in both CAFs and tumor cells. This pro-inflammatory milieu activates the IL-6/STAT3 axis, prompting CAFs to release increased amounts of IL-6 and the immunosuppressive enzyme indoleamine 2,3-dioxygenase (Cheng et al., 2016; Zheng et al., 2016). Consequently, CAFs fulfill a critical function in inducing dendritic cells (DCs) to adopt a regulatory state, reducing antigen-presenting capabilities, impairing the recruitment and activation of tumor-infiltrating lymphocytes (TILs), and enhancing the inhibition of myeloid-derived suppressor cells (MDSCs) through the fibroblast activation protein (FAP) and STAT3 axis. This intricate interaction participates in the formation of an inflammatory TME (Yang et al., 2016).
Several chemokines primarily secreted by CAFs assume pivotal roles in the interaction between the tumor and the immune microenvironment in CCA. CCL2 lures MDSCs to the TME, promoting CCA expansion (Lin et al., 2019). CXCL9 governs the attraction of tumor-infiltrating NK cells in CCA. Elevated CXCL9 levels correlates with improved overall survival after surgical resection (Fukuda et al., 2020). These discoveries indicate that targeting chemokines in the TME of CCA holds promise as a therapeutic approach to impede CCA growth and modulate the immune response.
As mentioned above, CAFs actively release a diverse repertoire of growth factors. PDGF-BB, secreted by myofibroblasts, it thwarts apoptosis triggered by TNF-α in CCA cells by activating PDGF receptor β (PDGFR β) in these cells (Fingas et al., 2011). HB-EGF, which is released by CAFs, functions as a ligand for EGFR. This activation of EGFR in CCA cells results in the stimulation of their in vitro migration and invasion. The use of neutralizing antibodies to block HB-EGF can effectively hinder the progression of CCA (Clapéron et al., 2013). Furthermore, after being stimulated by PDGF-D from cholangiocytes within the tumor, CAFs release VEGF-A and VEGF-C. These factors then attract and orchestrate the arrangement of lymphatic endothelial cells into vascular formations that are conducive to the intravasation of tumor cells. Navitoclax inhibits metastatic spread of the tumor in live organisms by inhibiting the release of VEGF-A/C by activated CAFs in CCA (Cadamuro et al., 2019).
In the carcinoma microenvironment, fibroblasts often adopt an altered phenotype, marked by increased expression of α-smooth muscle actin (α-SMA) and fibroblast activation protein (FAP). CAFs notably express α-SMA (Vaquero et al., 2020), and elevated α-SMA levels within the tumor stroma are linked to enhanced survival among patients diagnosed with CCA (Zhang et al., 2017; Itou et al., 2019). Moreover, periostin, an extracellular matrix protein synthesized by α-SMA-positive CAFs in iCCA, exhibits elevated expression compared to control tissues (Sirica et al., 2014). Increased periostin levels act as an indicator of malignant progression in CCA and are associated with a less favorable prognosis for patients (Manzanares et al., 2018).
Tumor cells exploit miRNAs carried by EVs as signaling agents that promote the establishment and activation of CAFs, subsequently influencing tumor cell behavior. Dysregulation of miRNAs is intimately connected to the activation and development of CAFs, impacting their tumor-supporting capabilities both within a controlled laboratory setting and within living organisms. MiRNAs within CAF-derived EVs can modulate the migration, infiltration, and metastasis of tumor cells, as well as the induction of drug resistance and determine aggressive cancer phenotypes. For instance, CAFs secrete exosomes enriched with miR-493-5p, promoting tumor progression. MiR-493-5p’s target expression, cocaine-amphetamine-regulated transcriptional precursor peptides, significantly correlates with intrahepatic cholangiocarcinoma (iCCA) prognosis (Toshida et al., 2023). Elevated miR-9-5p in EVs from iCCA cells induces IL-6 expression in CAFs, upregulates EZH2 in iCCA cells, and enhances tumor malignancy (Zhang et al., 2020). Conversely, downregulated miR-30e in human CCA cells can be partially restored by treating them with miR-30e-enriched EVs, leading to attenuated cell invasion and migration (Ota et al., 2018; Trifylli et al., 2023).
As vital constituents of the TME in CCA, CAFs, MSCs, and tumor cells utilize EVs as conduits for the transportation of diverse soluble molecules, including cytokines, chemokines, and growth factors (Figure 2). This intricate interplay serves as an imperative cog in the machinery of tumor progression. Through the exchange of these substances, the triad of cell types mutually propel and invigorate each other, instigating a cascade of events that promote inflammatory stimulation, angiogenesis, and the relentless expansion of tumorous growth. The complex interaction between CAFs and CCA cells, as well as immune cells, and MSCs continues to evolve, offering potential therapeutic targets. Given CAFs’ pivotal role in CCA progression, targeting them is considered a promising therapeutic strategy for CCA.
6 Immune cells and tumor cell-derived EVs act as modulators of tumor immunology
6.1 Macrophage
Tumor-associated macrophages (TAMs) are a diverse cell group within the TME, crucial for supporting CCA cell growth by releasing cytokines, chemokines, and growth factors, promoting angiogenesis, and suppressing specific immunity (Techasen et al., 2012; Raggi et al., 2017).
TAMs play a pivotal role in bridging the link in connecting the immune response to cancer by dampening adaptive immunity via cytokine secretion (Sica et al., 2008). Studies have revealed that TAMs release immunosuppressive cytokines, including IL-6 and IL-10, which hinder the protective functions of tumor-infiltrating lymphocytes (TILs), thus facilitating the process of epithelial-mesenchymal transition (EMT) and subsequent metastasis. Additionally, research has demonstrated the abundant secretion of OSM and IL-11, both belonging to the IL-6 cytokine family, by TAMs during inflammation and cancer. TAMs collaborate to advance iCCA progression through the OSM/IL-11/STAT3 signaling pathway (Zhou et al., 2021). Simultaneously, M2-polarized macrophages activated by iCCA support tumor expansion and invasiveness by means of EMT induced through the IL-10/STAT3 pathway. This provides a potential focus for therapeutic interventions aimed at iCCA (Yuan et al., 2020). TAMs exert their immunosuppressive effects indirectly by releasing chemokines that selectively attract T cell subsets deficient in cytotoxic capabilities. For instance, TAMs abundantly express chemokines like CCL17 and CCL22 that engage with the CCR4 receptor, primarily located on Th2 cells and Tregs, two T cell subsets with restricted anti-tumor capacities (Colombo and Mantovani, 2005). Moreover, chemokines within the tumor microenvironment have been demonstrated to enhance the recruitment of macrophages. The polarization of macrophages toward a tumor-promoting M2 state and increased TAM infiltration in CCA have been associated with poor prognosis and metastasis in CCA (Thanee et al., 2015; Kaneda et al., 2016). EVs derived from CAFs and tumor cells carrying CCL2 further support macrophage recruitment from circulating monocytes (Mitchem et al., 2013). Chemokines (e.g., CCL5, CCL7, CCL8, CXCL12) or cytokines such as VEGF, PDGF), and macrophage colony-stimulating factor (M-CSF) have also been confirmed to entice peripheral blood monocytes to the TME (Mantovani et al., 2006).
In the tumor stroma, many products released by macrophages can directly stimulate tumor cell proliferation, mobility, and metastatic spread. These products include epidermal growth factor (EGF), members of the FGF family, TGF-β, VEGF, PDGF, as well as various chemokines and cytokines. TAMs may play a role in promoting tumor advancement by aiding in the development of the stroma and the process of angiogenesis. They achieve this by releasing PDGF, which works in tandem with TGF-β produced by cancer cells. The accumulation of TAMs is associated with heightened angiogenesis and the secretion of angiogenic factors such as VEGF and PDGF. Additionally, research conducted in both controlled laboratory settings and living organisms has demonstrated that TAMs have the capacity to induce the expression of PD-L1 in both mouse and human cancer cells through the secretion of EGF (González and Falcón-Pérez, 2015).
EVs released by TAMs transport miRNAs to cells in the TME, contributing to pro-tumoral processes, including promoting cancer cell proliferation, migration, invasion, regulating immune cells to facilitate immune escape, and enhancing cancer cell resistance to anti-cancer drugs (Cocks et al., 2022). Notably, exosomal miR-183-5p downregulates the phosphatase and tension homolog (PTEN) expression, leading to heightened levels of phosphorylated AKT and PD-L1 expression in macrophages. Clinical data has indicated that elevated plasma exosomal miR-183-5p levels are associated with an unfavorable prognosis in iCCA patients following radical resection. Thus, exosomal miR-183-5p holds potential as a biomarker for predicting iCCA progression and as a target for developing therapeutic strategies to address immune tolerance features in iCCA (Shu et al., 2023).
6.2 Natural killer (NK) cell
As a crucial cell in the innate immune system, NK cells maintain homeostasis, resist viral incursions and thwart the survival or dissemination of malignantly altered cells. Preclinical investigations have indicated that the absence of NK cells or impaired NK cell function is linked to the advancement of tumors (Peng et al., 2017; Jun et al., 2019). The equilibrium between activation and inhibitory signals plays a crucial role in determining the activation status of NK cells. NK cells can be triggered into activation by HSP70 present in EVs, either in its soluble form or as a membrane-bound protein (Kim et al., 2006). The release of HSP70-containing EVs from tumor cells activates NK cells, which can reduce tumor growth by recognizing stress-induced NKG2D ligands on malignant cells (Elsner et al., 2010). The natural killer group 2D (NKG2D) is an activating receptor on NK cells, and it is responsible for targeting and eliminating tumor cells by binding to its ligand, NKG2D ligand (NKG2DL). Disruption or impairment of the NKG2D/NKG2DL axis contributes to the ability of tumors to evade detection by the immune system. Variations in the NKG2D receptor identified in individuals with PSC have been documented to heighten their vulnerability to CCA (Melum et al., 2008; Wadsworth et al., 2019). Additionally, elevated levels of NKG2D ligands in human CCA were linked to better disease-free and overall survival among patients (Tsukagoshi et al., 2016).
Human NK cells themselves constitutively release EVs. NK cell-derived EVs exhibit cytotoxicity to tumor cells and activated immune cells (Clayton et al., 2008; Lugini et al., 2012). Summarily, both EV derived from NK cells and EV released by stress cells or tumor cells can contribute to the regulation of the immune system by stimulating NK cells.
6.3 Dendritic cell (DC)
Within the TME, DCs occupy a pivotal role, serving as a linchpin in initiating and orchestrating both the innate and adaptive immune reactions. Antigens carried to DCs through EVs have the capability to trigger antigen-specific T cell reactions. The EV-delivered antigens released by tumor cells can suppress immune responses against the tumor, such as hindering the activation of T cells or DCs (Fabris et al., 2021). In CCA, the penetration of fully developed CD83+DCs is associated with the accumulation of CD4+/CD8+T cells in the surrounding area of the tumor. The existence of CD83+DCs cells was also linked to enhanced patient prognoses. On the contrary, immature CD1a DCs in the central part of tumors was correlated with the lack of CD4+/CD8+ T cells (Takagi et al., 2004). There are also studies indicating that FcεRI+ (a high-affinity immunoglobulin E receptor) monocytes and DCs in the bloodstream of individuals diagnosed with CCA were significantly decreased. These results suggest that DCs in CCA are impaired in their function and are unable to restrain the advancement of the tumor (Martín-Sierra et al., 2019).
EVs derived from tumors can modulate the immune responses of the host through a range of pathways, which includes promoting pro-inflammatory effects (Wang et al., 2022). Tumor-derived EVs induce monocytes to release pro-inflammatory cytokines. Research has shown that monocyte stimulated by EVs originating from cancer cells heightened the human leukocyte antigen DR (HLA-DR) expression, the production of reactive oxygen intermediates, mRNA accumulation, and the release of TNF, IL-10, IL-12 (Baj-Krzyworzeka et al., 2007). Crucially, EVs play a pivotal role in inducing liver inflammation, a hallmark of nearly all liver conditions, encompassing acute liver damage, chronic viral hepatitis, hepatocellular carcinoma (HCC), and cholangiopathy (Tkach et al., 2022). Various Clinical trials have seen the development of vaccines targeting DCs to augment cancer immunotherapy. Prior research has highlighted the feasibility of utilizing tumor-derived exosomes (Texs) as cell-free carriers for in situ DC activation in the TME (Huang et al., 2022). This approach, incorporating Tex-based delivery of stimulants and adjustable adjuvants to malignant cells may present a potential approach for treating CCA.
6.4 Mast cell
Mast cells have a critical function in innate immunity and the control of adaptive immunity through the secretion of various immunomodulatory mediators (Rodewald and Feyerabend, 2012). Mast cell-derived EVs contain immunomodulatory proteins, including MHC II, leukocyte function-associated antigen 1 (LFA-1), intercellular cell adhesion molecule-1 (ICAM-1), Heat Shock Proteins (HSP), and high-affinity IgE receptors (Carroll-Portillo et al., 2012). These EVs can home in on additional mast cells, provoke the maturation of DCs, transport antigens for cross-presentation, and activate B and T cells (Skokos et al., 2003). Within the TME, mast cells interact by engaging in direct cell-to-cell interactions with infiltrated immune cells, tumor cells, and the extracellular matrix (ECM), or through the release of a wide array of mediators that are capable of reshaping the TME. Mast cells play an active role in promoting angiogenesis by releasing both traditional proangiogenic factors such as VEGF, FGF-2, PDGF, and IL-6, and non-traditional proangiogenic factors, primarily proteases like tryptase and chymase. Moreover, mast cells facilitate tumor invasiveness by secreting a diverse array of matrix metalloproteinases (MMPs).
The process of interaction between mast cells and the TME of CCA can be initially observed in bile. Mast cell can infiltrate the liver during cholestasis and cause biliary damage. Within the model of bile duct ligation, increased Mast cells were observed around the damaged bile duct and increased biliary hyperplasia, liver injury, and fibrosis induced by bile duct ligation (Meadows et al., 2019). Prior investigations have likewise demonstrated that Mast cells may be involved in CCA progression by releasing histamine (HA) in CCA (Kennedy et al., 2018). Bile exosomal miR-182/183-5p is released by CCA cells targets the hydroxyprostaglandindehydrogenase (HPGD) in CCA cells and Mast cells and increases the production of Prostaglandin E2 (stimulates PTGER1), thereby promoting the proliferation, invasion, EMT of CCA. miR-182/183-5p also promotes angiogenesis by releasing VEGF-A expression to promote Mast cell release of VEGF-A. The study demonstrated for the first time that Mast cells constitute the predominant cell type within HPGD and also the target cells of bile exosomal miR-182/183-5p (Shu et al., 2023). Mast cells are key factors in the bile interaction of CCA, promoting CCA progression through the release of PGE2 and VEGF-A. The significant role of mast cells in the advancement, angiogenesis, and fibrogenesis of CCA suggests the possibility of treating CCA through the local administration of Mast cell stabilizers or anti-angiogenic drugs in the bile duct.
6.5 Tumor-infiltrating lymphocytes (TILs)
Tumor-infiltrating lymphocytes (TILs) consist of B lymphocytes, cytotoxic T cells (CD8+T), and T helper cells (CD4+T). The cellular makeup and molecular profiles of TILs reconfigure the CCA microenvironment, impacting the immune surveillance or evasion of cancer. Upon activation, T cells can generate immunomodulatory EVs carrying MHC, TCR, APO2 ligands, Fas ligand (FasL), and NKG2D ligands. These EVs have been shown to inhibit NK cytotoxicity (Alonso et al., 2011; Hedlund et al., 2011), block T cell stimulation (Busch et al., 2008), promote T cell apoptosis (Monleón et al., 2001), and diminish the stimulatory ability of antigen-presenting cells to activate T cells, thereby contributing to the suppression of the immune response (Xie et al., 2010). Research has demonstrated that extracellular matrix protein degradation via MMP-9 mediation may be heightened by CD8+ T cell-derived exosomes featuring membrane-bound FasL, consequently intensifying the invasive and metastatic potential of Fas+ tumor cells (Cai et al., 2012).
Although T cells constitutively release EV, T cell receptor (TCR) triggering and intracellular calcium stimulation increase EV secretion (Blanchard et al., 2002). In addition to immunosuppressive effects, EVs originating from T cells have been associated with the induction of T cell proliferation through a mechanism that relies on CCL5 (Wahlgren et al., 2012) and enhancement of immunogenicity is achieved by selectively modulating gene regulation within antigen-presenting cells (APCs) (Spaeth et al., 2009). The immune synapse interactions occur both at the interface of CD8+ cytotoxic T cells and tumor cells, as well as at the crossroads of T cells and APCs, is an efficient mechanism for the transfer of EVs (Mittelbrunn and Sánchez-Madrid, 2012). It was demonstrated that BMI1 suppresses CD8+ T cell chemokine recruitment by facilitating inhibitory H2A ubiquitination in CCA cells. BMI 1 is an indicator associated with an unfavorable prognosis for CCA. The unique exosomes containing BMI 1 promote the multiplication and metastasis of CCA via an autocrine/paracrine signaling pathway (Liu et al., 2022).
EVs secreted by various effector T cell subsets, including Th1, Th2, and Treg, exhibit unique miRNA profiles. Specific miRNAs in EVs derived from Treg cells inhibit pathogenic Th1 cells and inflammation (Seo et al., 2018). FoxP3 is a protein crucial for the formation and role of Tregs, and its downregulation in CCA cells leads to the reduction of TGF-β, thereby improving the survival of effector T cells (Ma et al., 2015). Similarly, the overexpression of FoxP3 within pancreatic ductal adenocarcinoma (PDAC) cells leads to the increased transcription of PD-L1 and the recruitment of Tregs, ultimately augmenting immune evasion by the tumor (Wang et al., 2020).
Adoptive cell therapy (ACT) is a form of cancer immunotherapy that harnesses the patient’s own immune cells to seek out and eliminate malignant cells. Initial discoveries showed that T cells were responsible for graft-versus-tumor responses. In the case of Tumor-Infiltrating Lymphocyte (TIL) ACT, TILs are extracted from surgically removed tumor tissue, expanded and enhanced outside the body, and subsequently reintroduced into the patient as therapeutic agents. ACT using TILs has displayed the capacity to induce significant tumor regression in various cancer types, including cholangiocarcinoma. In cancer immunotherapy, both CD8+ and CD4+ T cells play a role in combating tumors. Nonetheless, the field has predominantly focused on comprehending the anti-tumor cytotoxicity mediated by CD4+ T cells (Kumar et al., 2021). For instance, in TIL cultures from a cholangiocarcinoma patient, MHC class 2 (MHCII) antigen HLA-DQ O6-restricted CD4+ T cells that recognize the ERBB2IP mutation were identified (Tran et al., 2014). Several strategies are presently under clinical development for the identification of tumor-associated antigens and the creation of personalized Adoptive Cell Therapy (ACT) products to enhance the effectiveness of tumor control. One of the most advanced methods for personalizing Tumor-Infiltrating Lymphocyte (TIL) ACT is the identification and expansion of TILs that possess T cell receptors (TCRs) specifically tailored to target tumor neoantigens. Extracellular vesicles have the potential to act as antigen-presenting tools and could catalyze further progress in the development of ACT.
7 Extracellular vesicles and angiogenesis
The initiation and sustenance of tumor neovascularization are governed by a intricate network of interactions (Bikfalvi, 2003). EVs are implicated in various aspects of vascular regulation in malignancy. Tumor- and platelet-derived EVs are rich sources of angiogenic growth factors (VEGF, FGF) (Taraboletti et al., 2006) and pro-inflammatory cytokines [IL1β (Bianco et al., 2009)]. Growth factors and their receptors or adhesion molecules could expand neovascularization through synergistic effects with extracellular matrix (Carmeliet et al., 2009; Hong et al., 2009). These may contribute directly or indirectly to a pro-angiogenic intratumoral environment, possibly through several processes (Dolo et al., 2005; Ratajczak et al., 2006), including 1) cell-to-cell transfer of pro-angiogenic substances, 2) extracellular release of the pro-angiogenic contents of EV, or by 3) inducing pro-angiogenic alterations in gene expression following the interaction of vascular cells with EVs (Mostefai et al., 2008). For example, endothelial progenitor cells release EVs carrying mRNA that can be conveyed to local endothelial cells, inducing angiogenesis activation (Deregibus et al., 2007). Endothelial cells can likewise react to the transfer of mRNA from tumor cells or the uptake of EVs carrying the active EGFR oncoprotein (Al-Nedawi et al., 2009). In the latter scenario, endothelial cells display EGFR positivity both in vivo and in vitro, leading to the initiation of endogenous or autocrine angiogenic activity, such as VEGF production.
The primary role of VEGFs as specific growth factors for vascular endothelial cells is the induction of angiogenesis. VEGF is markedly upregulated in CCA and correlated with unfavorable patient outcomes (Vaeteewoottacharn et al., 2016). Platelets release EVs containing VEGF (Kim et al., 2004). Furthermore, it has been demonstrated that this factor is found within tumor-derived EVs and is exclusively released from EVs in a biologically active form under the acidic pH conditions of the TME (Taraboletti et al., 2006). VEGF-D modulates the function of stromal cells and facilitates tumor cell metastasis via lymphatic dissemination (Stacker et al., 2001). VEGF-C secreted by CAFs can enhance lymphatic endothelial cells’ (LECs) permeability, hence, promoting lymphoid infiltration and metastasis in CCA (Cadamuro et al., 2019). Single-cell transcriptome analysis of tumors from HCC patients, including 9 iCCA patients, revealed that VEGF is indispensable in intratumoral diversity (Ma et al., 2019). The results indicate that VEGF, induced by Hypoxia-Inducible Factor 1-α (HIF1α), orchestrates the manipulation of tumor endothelial cells, CAFs and TAMs, promoting tumor advancement. These findings suggest that blocking VEGF could potentially impede the progression and metastasis of CCA. Regorafenib, an oral multi-kinase inhibitor that targets VEGFR2, demonstrated a significant inhibition of CCA growth both in vitro and in vivo (Yeh et al., 2017).
Coordinated changes in promoting angiogenesis can be induced in multiple cells. One way to achieve this effect may be through the intercellular exchange of EVs (Al-Nedawi et al., 2008). When circular RNA circ-CCAC1, originating from EVs derived from CCA, was introduced into endothelial monolayers, the integrity of the endothelial barrier was disrupted and induced angiogenesis, leading to the tumorigenesis and metastasis of CCA tumors (Xu et al., 2021). Circular RNA circ-CCAC1 plays a pivotal role in the tumorigenesis and metastasis of CCA and could potentially serve as a significant biomarker and therapeutic target for CCA (Trifylli et al., 2023).
8 Discussion
EVs play a crucial role in the growth and advancement of cancer, which encompasses the establishment of the tumor microenvironment, angiogenesis, and the stimulation of tumor growth and invasion. Disrupting the generation, emission, and absorption of EVs at various stages can be considered a viable therapeutic strategy for cancer. Among them, intervention in the production of ceramide is the most commonly employed approach to reduce the production of exosomes in tumor. Suppression of ceramide production using myriocin, a selective inhibitor of serine palmitoyltransferase, diminished the pro-inflammatory properties of EVs derived from iCCA, inhibition of neutral type II sphingomyelinase (nSMase2), which accountable for the synthesis of ceramide, and its inhibitor GW4869 can reduce exocrine secretion (Trajkovic et al., 2008; Singh et al., 2014) and make cancer cells sensitive to chemotherapy (Richards et al., 2017). Notably, GW4869 inhibited the migratory ability of CCA cells (Haga et al., 2015). Obstructing or eliminating cancer-generated EVs through apheresis utilizing specialized devices represents a promising therapeutic method. In the meantime, EVs are also a strong contender for delivering novel anticancer proteins, drugs, or cancer vaccines.
As an antigen carrier, EV provides a new method for exploring personalized immunotherapy for cholangiocarcinoma. Among them, immunotherapy is the most widely studied. Exosomes derived from AFP-expressing dendritic cells (DCs) (DEXAFP) induced specific and robust immune responses against the antigen. They caused significant tumor growth retardation and prolonged survival in HCC tumor mice induced by ectopic, in situ, or carcinogens. In hepatocellular carcinoma (HCC) mice subjected to DEXAFP treatment, the TME showed notable enhancements. This was evident through a substantial increase in the count of CD8+ T lymphocytes expressing IFN-γ, elevated levels of IFN-γ and IL-2, a reduction in the quantity of CD25+ Foxp3+ Treg cells, diminished levels of IL-10, and a decrease in the presence of transforming TGF-β at the tumor sites (Rao et al., 2016). DC-derived exosomes offer innovative avenues for the development of cancer immunotherapy vaccines (Lu et al., 2017). Apart from its involvement in EV-based therapy, what is contented in EVs can serve as an indicator of response to immunotherapy (Zuo et al., 2020). Therefore, the release of EVs from tumor cells or circulating exosomes presents a novel therapeutic target. Exosomes can serve as a vehicle for the targeted transport of medications, therapeutic compounds, or gene therapy materials to tumors.
Moreover, exosomal molecules can serve as biomarkers for the early detection and diagnosis of diseases, as well as for assessing prognosis and predicting therapeutic outcomes based on the molecular signatures of exosomes (Zhang and Grizzle, 2011). Vesicles displaying exosome-like traits have been successfully isolated from a range of bodily fluids, including blood (Caby et al., 2005), urine (Huebner et al., 2015), bile (Masyuk et al., 2010), malignant effusion (Andre et al., 2002). Recent research findings have indicated that miRNA-based panels exhibit specificity for CCA and are derived from miRNAs isolated from human biliary EVs, thus indicating the potential utility of biliary EVs as a diagnostic approach (Arbelaiz et al., 2017; Sirica et al., 2019; Lapitz et al., 2020). In summary, these investigations suggest that exosome proteins can be considered early diagnostic biomarkers rather than just proteins in the tumor itself. Currently, the most significant obstacle to the effective utilization of EVs as a diagnostic and prognostic tool lies in the considerable inter-individual variability in protein content within various bodily fluids, such as urine and blood. Additionally, the requirement for standardized procedures for preserving, purifying, and examining vesicles from diverse bodily fluids imposes notable constraints. This factor implies that the existing gold standard technique for EV isolation is not applicable in clinical contexts (González and Falcón-Pérez, 2015). Extensive research is still needed before some enter routine clinical practice.
Author contributions
NZ: Investigation, Writing–original draft, Writing–review and editing. LS: Investigation, Writing–original draft, Writing–review and editing. ZL: Investigation, Writing–review and editing. AS: Investigation, Writing–review and editing. LZ: Writing–review and editing. SH: Writing–review and editing. GS: Writing–review and editing. ZY: Writing–review and editing. YS: Writing–review and editing. FH: Writing–review and editing. YT: Investigation, Supervision, Writing–review and editing. ZZ: Writing–review and editing.
Funding
The author(s) declare financial support was received for the research, authorship, and/or publication of this article. Shandong Province Key R&D Program (Major Scientific Innovation Projects, 2021CXGC011105), Key Research and Development Program of Shandong Province (Grant No. 2019GSF108254), Clinical Research Foundation of Shandong University (Grant No. 2020SDUCRCA018), Shandong Province Natural Science Foundation (Grant No. ZR2021QH079), Shandong Province Natural Science Foundation Youth Program (Grant No. ZR2023QH535), and the National Natural Science Foundation of China (Grant No. 82203766).
Conflict of interest
The authors declare that the research was conducted in the absence of any commercial or financial relationships that could be construed as a potential conflict of interest.
Publisher’s note
All claims expressed in this article are solely those of the authors and do not necessarily represent those of their affiliated organizations, or those of the publisher, the editors and the reviewers. Any product that may be evaluated in this article, or claim that may be made by its manufacturer, is not guaranteed or endorsed by the publisher.
References
Akers, J. C., Gonda, D., Kim, R., Carter, B. S., and Chen, C. C. (2013). Biogenesis of extracellular vesicles (EV): exosomes, microvesicles, retrovirus-like vesicles, and apoptotic bodies. J. neuro-oncology 113 (1), 1–11. doi:10.1007/s11060-013-1084-8
Al-Nedawi, K., Meehan, B., Kerbel, R. S., Allison, A. C., and Rak, J. (2009). Endothelial expression of autocrine VEGF upon the uptake of tumor-derived microvesicles containing oncogenic EGFR. Proc. Natl. Acad. Sci. U. S. A. 106 (10), 3794–3799. doi:10.1073/pnas.0804543106
Al-Nedawi, K., Meehan, B., Micallef, J., Lhotak, V., May, L., Guha, A., et al. (2008). Intercellular transfer of the oncogenic receptor EGFRvIII by microvesicles derived from tumour cells. Nat. Cell Biol. 10 (5), 619–624. doi:10.1038/ncb1725
Alonso, R., Mazzeo, C., Rodriguez, M. C., Marsh, M., Fraile-Ramos, A., Calvo, V., et al. (2011). Diacylglycerol kinase α regulates the formation and polarisation of mature multivesicular bodies involved in the secretion of Fas ligand-containing exosomes in T lymphocytes. Cell death Differ. 18 (7), 1161–1173. doi:10.1038/cdd.2010.184
Andre, F., Schartz, N. E. C., Movassagh, M., Flament, C., Pautier, P., Morice, P., et al. (2002). Malignant effusions and immunogenic tumour-derived exosomes. Lancet (London, Engl. 360 (9329), 295–305. doi:10.1016/S0140-6736(02)09552-1
Arbelaiz, A., Azkargorta, M., Krawczyk, M., Santos-Laso, A., Lapitz, A., Perugorria, M. J., et al. (2017). Serum extracellular vesicles contain protein biomarkers for primary sclerosing cholangitis and cholangiocarcinoma. Hepatol. Baltim. Md 66 (4), 1125–1143. doi:10.1002/hep.29291
Baghban, R., Roshangar, L., Jahanban-Esfahlan, R., Seidi, K., Ebrahimi-Kalan, A., Jaymand, M., et al. (2020). Tumor microenvironment complexity and therapeutic implications at a glance. Cell Commun. Signal. CCS 18 (1), 59. doi:10.1186/s12964-020-0530-4
Baj-Krzyworzeka, M., Szatanek, R., Weglarczyk, K., Baran, J., and Zembala, M. (2007). Tumour-derived microvesicles modulate biological activity of human monocytes. Immunol. Lett. 113 (2), 76–82. doi:10.1016/j.imlet.2007.07.014
Balaj, L., Lessard, R., Dai, L., Cho, Y.-J., Pomeroy, S. L., Breakefield, X. O., et al. (2011). Tumour microvesicles contain retrotransposon elements and amplified oncogene sequences. Nat. Commun. 2, 180. doi:10.1038/ncomms1180
Balakrishnan, K., Burger, J. A., Quiroga, M. P., Henneberg, M., Ayres, M. L., Wierda, W. G., et al. (2010). Influence of bone marrow stromal microenvironment on forodesine-induced responses in CLL primary cells. Blood 116 (7), 1083–1091. doi:10.1182/blood-2009-10-246199
Banales, J. M., Cardinale, V., Carpino, G., Marzioni, M., Andersen, J. B., Invernizzi, P., et al. (2016). Expert consensus document: cholangiocarcinoma: current knowledge and future perspectives consensus statement from the European Network for the Study of Cholangiocarcinoma (ENS-CCA). Nat. Rev. Gastroenterology hepatology 13 (5), 261–280. doi:10.1038/nrgastro.2016.51
Banales, J. M., Marin, J. J. G., Lamarca, A., Rodrigues, P. M., Khan, S. A., Roberts, L. R., et al. (2020). Cholangiocarcinoma 2020: the next horizon in mechanisms and management. Nat. Rev. Gastroenterology hepatology 17 (9), 557–588. doi:10.1038/s41575-020-0310-z
Bianco, F., Perrotta, C., Novellino, L., Francolini, M., Riganti, L., Menna, E., et al. (2009). Acid sphingomyelinase activity triggers microparticle release from glial cells. EMBO J. 28 (8), 1043–1054. doi:10.1038/emboj.2009.45
Blanchard, N., Lankar, D., Faure, F., Regnault, A., Dumont, C., Raposo, G., et al. (2002). TCR activation of human T cells induces the production of exosomes bearing the TCR/CD3/zeta complex. J. Immunol. 168 (7), 3235–3241. doi:10.4049/jimmunol.168.7.3235
Blechacz, B., Komuta, M., Roskams, T., and Gores, G. J. (2011). Clinical diagnosis and staging of cholangiocarcinoma. Nat. Rev. Gastroenterology hepatology 8 (9), 512–522. doi:10.1038/nrgastro.2011.131
Bouvy, C., Gheldof, D., Chatelain, C., Mullier, F., and Dogné, J.-M. (2014). Contributing role of extracellular vesicles on vascular endothelium haemostatic balance in cancer. J. Extracell. vesicles 3. doi:10.3402/jev.v3.24400
Braconi, C., and Patel, T. (2010). Cholangiocarcinoma: new insights into disease pathogenesis and biology. Infect. Dis. Clin. N. Am. 24 (4), 871–884. doi:10.1016/j.idc.2010.07.006
Braconi, C., Swenson, E., Kogure, T., Huang, N., and Patel, T. (2010). Targeting the IL-6 dependent phenotype can identify novel therapies for cholangiocarcinoma. PloS one 5 (12), e15195. doi:10.1371/journal.pone.0015195
Busch, A., Quast, T., Keller, S., Kolanus, W., Knolle, P., Altevogt, P., et al. (2008). Transfer of T cell surface molecules to dendritic cells upon CD4+ T cell priming involves two distinct mechanisms. J. Immunol. 181 (6), 3965–3973. doi:10.4049/jimmunol.181.6.3965
Caby, M.-P., Lankar, D., Vincendeau-Scherrer, C., Raposo, G., and Bonnerot, C. (2005). Exosomal-like vesicles are present in human blood plasma. Int. Immunol. 17 (7), 879–887. doi:10.1093/intimm/dxh267
Cadamuro, M., Brivio, S., Mertens, J., Vismara, M., Moncsek, A., Milani, C., et al. (2019). Platelet-derived growth factor-D enables liver myofibroblasts to promote tumor lymphangiogenesis in cholangiocarcinoma. J. hepatology 70 (4), 700–709. doi:10.1016/j.jhep.2018.12.004
Cadamuro, M., Nardo, G., Indraccolo, S., Dall'olmo, L., Sambado, L., Moserle, L., et al. (2013). Platelet-derived growth factor-D and Rho GTPases regulate recruitment of cancer-associated fibroblasts in cholangiocarcinoma. Hepatol. Baltim. Md 58 (3), 1042–1053. doi:10.1002/hep.26384
Cai, Z., Yang, F., Yu, L., Yu, Z., Jiang, L., Wang, Q., et al. (2012). Activated T cell exosomes promote tumor invasion via Fas signaling pathway. J. Immunol. 188 (12), 5954–5961. doi:10.4049/jimmunol.1103466
Carmeliet, P., De Smet, F., Loges, S., and Mazzone, M. (2009). Branching morphogenesis and antiangiogenesis candidates: tip cells lead the way. Nat. Rev. Clin. Oncol. 6 (6), 315–326. doi:10.1038/nrclinonc.2009.64
Carroll-Portillo, A., Surviladze, Z., Cambi, A., Lidke, D. S., and Wilson, B. S. (2012). Mast cell synapses and exosomes: membrane contacts for information exchange. Front. Immunol. 3, 46. doi:10.3389/fimmu.2012.00046
Chaiyadet, S., Sotillo, J., Smout, M., Cantacessi, C., Jones, M. K., Johnson, M. S., et al. (2015). Carcinogenic liver fluke secretes extracellular vesicles that promote cholangiocytes to adopt a tumorigenic phenotype. J. Infect. Dis. 212 (10), 1636–1645. doi:10.1093/infdis/jiv291
Chalmin, F., Ladoire, S., Mignot, G., Vincent, J., Bruchard, M., Remy-Martin, J.-P., et al. (2010). Membrane-associated Hsp72 from tumor-derived exosomes mediates STAT3-dependent immunosuppressive function of mouse and human myeloid-derived suppressor cells. J. Clin. investigation 120 (2), 457–471. doi:10.1172/JCI40483
Chen, T., Guo, J., Yang, M., Zhu, X., and Cao, X. (2011). Chemokine-containing exosomes are released from heat-stressed tumor cells via lipid raft-dependent pathway and act as efficient tumor vaccine. J. Immunol. 186 (4), 2219–2228. doi:10.4049/jimmunol.1002991
Chen, W.-x., Liu, X.-m., Lv, M.-m., Chen, L., Zhao, J.-h., Zhong, S.-l., et al. (2014). Exosomes from drug-resistant breast cancer cells transmit chemoresistance by a horizontal transfer of microRNAs. PloS one 9 (4), e95240. doi:10.1371/journal.pone.0095240
Cheng, J. T., Deng, Y. N., Yi, H. M., Wang, G. Y., Fu, B. S., Chen, W. J., et al. (2016). Hepatic carcinoma-associated fibroblasts induce Ido-producing regulatory dendritic cells through IL-6-mediated STAT3 activation. Oncogenesis 5 (2), e198. doi:10.1038/oncsis.2016.7
Clapéron, A., Mergey, M., Aoudjehane, L., Ho-Bouldoires, T. H. N., Wendum, D., Prignon, A., et al. (2013). Hepatic myofibroblasts promote the progression of human cholangiocarcinoma through activation of epidermal growth factor receptor. Hepatol. Baltim. Md 58 (6), 2001–2011. doi:10.1002/hep.26585
Clapéron, A., Mergey, M., Nguyen Ho-Bouldoires, T. H., Vignjevic, D., Wendum, D., Chrétien, Y., et al. (2014). EGF/EGFR axis contributes to the progression of cholangiocarcinoma through the induction of an epithelial-mesenchymal transition. J. hepatology 61 (2), 325–332. doi:10.1016/j.jhep.2014.03.033
Clayton, A., Mitchell, J. P., Court, J., Linnane, S., Mason, M. D., and Tabi, Z. (2008). Human tumor-derived exosomes down-modulate NKG2D expression. J. Immunol. 180 (11), 7249–7258. doi:10.4049/jimmunol.180.11.7249
Cocks, A., Del Vecchio, F., Martinez-Rodriguez, V., Schukking, M., and Fabbri, M. (2022). Pro-tumoral functions of tumor-associated macrophage EV-miRNA. Seminars cancer Biol. 86 (1), 58–63. doi:10.1016/j.semcancer.2021.08.001
Colombo, M., Raposo, G., and Théry, C. (2014). Biogenesis, secretion, and intercellular interactions of exosomes and other extracellular vesicles. Annu. Rev. Cell Dev. Biol. 30, 255–289. doi:10.1146/annurev-cellbio-101512-122326
Colombo, M. P., and Mantovani, A. (2005). Targeting myelomonocytic cells to revert inflammation-dependent cancer promotion. Cancer Res. 65 (20), 9113–9116. doi:10.1158/0008-5472.CAN-05-2714
De Bruyn, C., Najar, M., Raicevic, G., Meuleman, N., Pieters, K., Stamatopoulos, B., et al. (2011). A rapid, simple, and reproducible method for the isolation of mesenchymal stromal cells from Wharton's jelly without enzymatic treatment. Stem Cells Dev. 20 (3), 547–557. doi:10.1089/scd.2010.0260
Deng, Y., Cheng, J., Fu, B., Liu, W., Chen, G., Zhang, Q., et al. (2017). Hepatic carcinoma-associated fibroblasts enhance immune suppression by facilitating the generation of myeloid-derived suppressor cells. Oncogene 36 (8), 1090–1101. doi:10.1038/onc.2016.273
Deregibus, M. C., Cantaluppi, V., Calogero, R., Lo Iacono, M., Tetta, C., Biancone, L., et al. (2007). Endothelial progenitor cell derived microvesicles activate an angiogenic program in endothelial cells by a horizontal transfer of mRNA. Blood 110 (7), 2440–2448. doi:10.1182/blood-2007-03-078709
De Wever, O., Van Bockstal, M., Mareel, M., Hendrix, A., and Bracke, M. (2014). Carcinoma-associated fibroblasts provide operational flexibility in metastasis. Seminars cancer Biol. 25, 33–46. doi:10.1016/j.semcancer.2013.12.009
Dolo, V., D'Ascenzo, S., Giusti, I., Millimaggi, D., Taraboletti, G., and Pavan, A. (2005). Shedding of membrane vesicles by tumor and endothelial cells. Italian J. Anat. embryology = Archivio italiano di anatomia ed embriologia 110 (2), 127–133.
Dutta, S., Reamtong, O., Panvongsa, W., Kitdumrongthum, S., Janpipatkul, K., Sangvanich, P., et al. (2015). Proteomics profiling of cholangiocarcinoma exosomes: a potential role of oncogenic protein transferring in cancer progression. Biochimica biophysica acta 1852 (9), 1989–1999. doi:10.1016/j.bbadis.2015.06.024
Dwyer, B. J., Jarman, E. J., Gogoi-Tiwari, J., Ferreira-Gonzalez, S., Boulter, L., Guest, R. V., et al. (2021). TWEAK/Fn14 signalling promotes cholangiocarcinoma niche formation and progression. J. hepatology 74 (4), 860–872. doi:10.1016/j.jhep.2020.11.018
Elsner, L., Flügge, P. F., Lozano, J., Muppala, V., Eiz-Vesper, B., Demiroglu, S. Y., et al. (2010). The endogenous danger signals HSP70 and MICA cooperate in the activation of cytotoxic effector functions of NK cells. J. Cell. Mol. Med. 14 (4), 992–1002. doi:10.1111/j.1582-4934.2009.00677.x
Fabris, L., Sato, K., Alpini, G., and Strazzabosco, M. (2021). The tumor microenvironment in cholangiocarcinoma progression. Hepatology 73 (1), 75–85. doi:10.1002/hep.31410
Fingas, C. D., Bronk, S. F., Werneburg, N. W., Mott, J. L., Guicciardi, M. E., Cazanave, S. C., et al. (2011). Myofibroblast-derived PDGF-BB promotes Hedgehog survival signaling in cholangiocarcinoma cells. Hepatol. Baltim. Md 54 (6), 2076–2088. doi:10.1002/hep.24588
Fukuda, Y., Asaoka, T., Eguchi, H., Yokota, Y., Kubo, M., Kinoshita, M., et al. (2020). Endogenous CXCL9 affects prognosis by regulating tumor-infiltrating natural killer cells in intrahepatic cholangiocarcinoma. Cancer Sci. 111 (2), 323–333. doi:10.1111/cas.14267
Fullár, A., Kovalszky, I., Bitsche, M., Romani, A., Schartinger, V. H., Sprinzl, G. M., et al. (2012). Tumor cell and carcinoma-associated fibroblast interaction regulates matrix metalloproteinases and their inhibitors in oral squamous cell carcinoma. Exp. Cell Res. 318 (13), 1517–1527. doi:10.1016/j.yexcr.2012.03.023
González, E., and Falcón-Pérez, J. M. (2015). Cell-derived extracellular vesicles as a platform to identify low-invasive disease biomarkers. Expert Rev. Mol. diagnostics 15 (7), 907–923. doi:10.1586/14737159.2015.1043272
Haga, H., Yan, I. K., Takahashi, K., Wood, J., Zubair, A., and Patel, T. (2015). Tumour cell-derived extracellular vesicles interact with mesenchymal stem cells to modulate the microenvironment and enhance cholangiocarcinoma growth. J. Extracell. Vesicles 4, 24900. doi:10.3402/jev.v4.24900
Haghnegahdar, H., Du, J., Wang, D., Strieter, R. M., Burdick, M. D., Nanney, L. B., et al. (2000). The tumorigenic and angiogenic effects of MGSA/GRO proteins in melanoma. J. Leukoc. Biol. 67 (1), 53–62. doi:10.1002/jlb.67.1.53
Hargrove, L., Kennedy, L., Demieville, J., Jones, H., Meng, F., DeMorrow, S., et al. (2017). Bile duct ligation-induced biliary hyperplasia, hepatic injury, and fibrosis are reduced in mast cell-deficient KitW-sh mice. Hepatol. Baltim. Md 65 (6), 1991–2004. doi:10.1002/hep.29079
Hedlund, M., Nagaeva, O., Kargl, D., Baranov, V., and Mincheva-Nilsson, L. (2011). Thermal- and oxidative stress causes enhanced release of NKG2D ligand-bearing immunosuppressive exosomes in leukemia/lymphoma T and B cells. PloS one 6 (2), e16899. doi:10.1371/journal.pone.0016899
Hong, B. S., Cho, J.-H., Kim, H., Choi, E.-J., Rho, S., Kim, J., et al. (2009). Colorectal cancer cell-derived microvesicles are enriched in cell cycle-related mRNAs that promote proliferation of endothelial cells. BMC Genomics 10, 556. doi:10.1186/1471-2164-10-556
Hoshino, A., Costa-Silva, B., Shen, T.-L., Rodrigues, G., Hashimoto, A., Tesic Mark, M., et al. (2015). Tumour exosome integrins determine organotropic metastasis. Nature 527 (7578), 329–335. doi:10.1038/nature15756
Huang, L., Rong, Y., Tang, X., Yi, K., Qi, P., Hou, J., et al. (2022). Engineered exosomes as an in situ DC-primed vaccine to boost antitumor immunity in breast cancer. Mol. Cancer 21 (1), 45. doi:10.1186/s12943-022-01515-x
Huang, W. H., Chang, M. C., Tsai, K. S., Hung, M. C., Chen, H. L., and Hung, S. C. (2013). Mesenchymal stem cells promote growth and angiogenesis of tumors in mice. Oncogene 32 (37), 4343–4354. doi:10.1038/onc.2012.458
Huebner, A. R., Somparn, P., Benjachat, T., Leelahavanichkul, A., Avihingsanon, Y., Fenton, R. A., et al. (2015). Exosomes in urine biomarker discovery. Adv. Exp. Med. Biol. 845, 43–58. doi:10.1007/978-94-017-9523-4_5
Itou, R. A., Uyama, N., Hirota, S., Kawada, N., Wu, S., Miyashita, S., et al. (2019). Immunohistochemical characterization of cancer-associated fibroblasts at the primary sites and in the metastatic lymph nodes of human intrahepatic cholangiocarcinoma. Hum. Pathol. 83, 77–89. doi:10.1016/j.humpath.2018.08.016
Janowska-Wieczorek, A., Wysoczynski, M., Kijowski, J., Marquez-Curtis, L., Machalinski, B., Ratajczak, J., et al. (2005). Microvesicles derived from activated platelets induce metastasis and angiogenesis in lung cancer. Int. J. cancer 113 (5), 752–760. doi:10.1002/ijc.20657
Jin, M.-Z., and Jin, W.-L. (2020). The updated landscape of tumor microenvironment and drug repurposing. Signal Transduct. Target. Ther. 5 (1), 166. doi:10.1038/s41392-020-00280-x
Jun, E., Song, A. Y., Choi, J.-W., Lee, H. H., Kim, M.-Y., Ko, D.-H., et al. (2019). Progressive impairment of NK cell cytotoxic degranulation is associated with TGF-β1 deregulation and disease progression in pancreatic cancer. Front. Immunol. 10, 1354. doi:10.3389/fimmu.2019.01354
Kahlert, C., Melo, S. A., Protopopov, A., Tang, J., Seth, S., Koch, M., et al. (2014). Identification of double-stranded genomic DNA spanning all chromosomes with mutated KRAS and p53 DNA in the serum exosomes of patients with pancreatic cancer. J. Biol. Chem. 289 (7), 3869–3875. doi:10.1074/jbc.C113.532267
Kaneda, M. M., Cappello, P., Nguyen, A. V., Ralainirina, N., Hardamon, C. R., Foubert, P., et al. (2016). Macrophage PI3Kγ drives pancreatic ductal adenocarcinoma progression. Cancer Discov. 6 (8), 870–885. doi:10.1158/2159-8290.CD-15-1346
Kennedy, L., Hargrove, L., Demieville, J., Karstens, W., Jones, H., DeMorrow, S., et al. (2018). Blocking H1/H2 histamine receptors inhibits damage/fibrosis in Mdr2 mice and human cholangiocarcinoma tumorigenesis. Hepatol. Baltim. Md 68 (3), 1042–1056. doi:10.1002/hep.29898
Kerkelä, E., Hakkarainen, T., Mäkelä, T., Raki, M., Kambur, O., Kilpinen, L., et al. (2013). Transient proteolytic modification of mesenchymal stromal cells increases lung clearance rate and targeting to injured tissue. Stem cells Transl. Med. 2 (7), 510–520. doi:10.5966/sctm.2012-0187
Kim, C. W., Lee, H. M., Lee, T. H., Kang, C., Kleinman, H. K., and Gho, Y. S. (2002). Extracellular membrane vesicles from tumor cells promote angiogenesis via sphingomyelin. Cancer Res. 62 (21), 6312–6317.
Kim, H. K., Song, K. S., Chung, J.-H., Lee, K. R., and Lee, S.-N. (2004). Platelet microparticles induce angiogenesis in vitro. Br. J. Haematol. 124 (3), 376–384. doi:10.1046/j.1365-2141.2003.04773.x
Kim, H. P., Morse, D., and Choi, A. M. K. (2006). Heat-shock proteins: new keys to the development of cytoprotective therapies. Expert Opin. Ther. targets 10 (5), 759–769. doi:10.1517/14728222.10.5.759
Komi, D. E. A., and Redegeld, F. A. (2020). Role of mast cells in shaping the tumor microenvironment. Clin. Rev. allergy & Immunol. 58 (3), 313–325. doi:10.1007/s12016-019-08753-w
Kumar, A., Watkins, R., and Vilgelm, A. E. (2021). Cell therapy with TILs: training and taming T cells to fight cancer. Front. Immunol. 12, 690499. doi:10.3389/fimmu.2021.690499
Lapitz, A., Arbelaiz, A., O'Rourke, C. J., Lavin, J. L., Casta, A. L., Ibarra, C., et al. (2020). Patients with cholangiocarcinoma present specific RNA profiles in serum and urine extracellular vesicles mirroring the tumor expression: novel liquid biopsy biomarkers for disease diagnosis. Cells 9 (3), 721. doi:10.3390/cells9030721
Lemoinne, S., Thabut, D., Housset, C., Moreau, R., Valla, D., Boulanger, C. M., et al. (2014). The emerging roles of microvesicles in liver diseases. Nat. Rev. Gastroenterology hepatology 11 (6), 350–361. doi:10.1038/nrgastro.2014.7
Liechty, K. W., MacKenzie, T. C., Shaaban, A. F., Radu, A., Moseley, A. M., Deans, R., et al. (2000). Human mesenchymal stem cells engraft and demonstrate site-specific differentiation after in utero transplantation in sheep. Nat. Med. 6 (11), 1282–1286. doi:10.1038/81395
Lin, Y., Li, B., Yang, X., Cai, Q., Liu, W., Tian, M., et al. (2019). Fibroblastic FAP promotes intrahepatic cholangiocarcinoma growth via MDSCs recruitment. Neoplasia (New York, N.Y.) 21 (12), 1133–1142. doi:10.1016/j.neo.2019.10.005
Lindoso, R. S., Collino, F., and Vieyra, A. (2017). Extracellular vesicles as regulators of tumor fate: crosstalk among cancer stem cells, tumor cells and mesenchymal stem cells. Stem Cell Investig. 4, 75. doi:10.21037/sci.2017.08.08
Liu, Y.-J., Li, K., Yang, L., Tang, S.-T., Wang, X.-X., Cao, G.-Q., et al. (2015). Dendritic cells regulate Treg-Th17 Axis in obstructive phase of bile duct injury in murine biliary atresia. PloS One 10 (9), e0136214. doi:10.1371/journal.pone.0136214
Liu, Z., Hu, C., Zheng, L., Liu, J., Li, K., Li, X., et al. (2022). BMI1 promotes cholangiocarcinoma progression and correlates with antitumor immunity in an exosome-dependent manner. Cell. Mol. life Sci. CMLS 79 (9), 469. doi:10.1007/s00018-022-04500-1
Lu, Z., Zuo, B., Jing, R., Gao, X., Rao, Q., Liu, Z., et al. (2017). Dendritic cell-derived exosomes elicit tumor regression in autochthonous hepatocellular carcinoma mouse models. J. hepatology 67 (4), 739–748. doi:10.1016/j.jhep.2017.05.019
Lugini, L., Cecchetti, S., Huber, V., Luciani, F., Macchia, G., Spadaro, F., et al. (2012). Immune surveillance properties of human NK cell-derived exosomes. J. Immunol. 189 (6), 2833–2842. doi:10.4049/jimmunol.1101988
Ma, C., Peng, C., Lu, X., Ding, X., Zhang, S., Zou, X., et al. (2015). Downregulation of FOXP3 inhibits invasion and immune escape in cholangiocarcinoma. Biochem. biophysical Res. Commun. 458 (2), 234–239. doi:10.1016/j.bbrc.2015.01.067
Ma, L., Hernandez, M. O., Zhao, Y., Mehta, M., Tran, B., Kelly, M., et al. (2019). Tumor cell biodiversity drives microenvironmental reprogramming in liver cancer. Cancer Cell 36 (4), 418–430. doi:10.1016/j.ccell.2019.08.007
Mantovani, A., Schioppa, T., Porta, C., Allavena, P., and Sica, A. (2006). Role of tumor-associated macrophages in tumor progression and invasion. Cancer metastasis Rev. 25 (3), 315–322. doi:10.1007/s10555-006-9001-7
Manzanares, M. Á., Campbell, D. J. W., Maldonado, G. T., and Sirica, A. E. (2018). Overexpression of periostin and distinct mesothelin forms predict malignant progression in a rat cholangiocarcinoma model. Hepatol. Commun. 2 (2), 155–172. doi:10.1002/hep4.1131
Martín-Sierra, C., Martins, R., Laranjeira, P., Abrantes, A. M., Oliveira, R. C., Tralhão, J. G., et al. (2019). Functional impairment of circulating FcεRI+ monocytes and myeloid dendritic cells in hepatocellular carcinoma and cholangiocarcinoma patients. Cytom. Part B, Clin. Cytom. 96 (6), 490–495. doi:10.1002/cyto.b.21777
Masyuk, A. I., Huang, B. Q., Ward, C. J., Gradilone, S. A., Banales, J. M., Masyuk, T. V., et al. (2010). Biliary exosomes influence cholangiocyte regulatory mechanisms and proliferation through interaction with primary cilia. Am. J. physiology. Gastrointest. liver physiology 299 (4), G990–G999. doi:10.1152/ajpgi.00093.2010
McAndrews, K. M., McGrail, D. J., Ravikumar, N., and Dawson, M. R. (2015). Mesenchymal stem cells induce directional migration of invasive breast cancer cells through TGF-β. Sci. Rep. 5, 16941. doi:10.1038/srep16941
Meadows, V., Kennedy, L., Hargrove, L., Demieville, J., Meng, F., Virani, S., et al. (2019). Downregulation of hepatic stem cell factor by Vivo-Morpholino treatment inhibits mast cell migration and decreases biliary damage/senescence and liver fibrosis in Mdr2 mice. Biochimica biophysica acta. Mol. basis Dis. 1865 (12), 165557. doi:10.1016/j.bbadis.2019.165557
Mele, V., Muraro, M. G., Calabrese, D., Pfaff, D., Amatruda, N., Amicarella, F., et al. (2014). Mesenchymal stromal cells induce epithelial-to-mesenchymal transition in human colorectal cancer cells through the expression of surface-bound TGF-β. Int. J. cancer 134 (11), 2583–2594. doi:10.1002/ijc.28598
Melo, S. A., Luecke, L. B., Kahlert, C., Fernandez, A. F., Gammon, S. T., Kaye, J., et al. (2015). Glypican-1 identifies cancer exosomes and detects early pancreatic cancer. Nature 523 (7559), 177–182. doi:10.1038/nature14581
Melum, E., Karlsen, T. H., Schrumpf, E., Bergquist, A., Thorsby, E., Boberg, K. M., et al. (2008). Cholangiocarcinoma in primary sclerosing cholangitis is associated with NKG2D polymorphisms. Hepatol. Baltim. Md 47 (1), 90–96. doi:10.1002/hep.21964
Melzer, C., Yang, Y., and Hass, R. (2016). Interaction of MSC with tumor cells. Cell Commun. Signal. CCS 14 (1), 20. doi:10.1186/s12964-016-0143-0
Millimaggi, D., Mari, M., D'Ascenzo, S., Carosa, E., Jannini, E. A., Zucker, S., et al. (2007). Tumor vesicle-associated CD147 modulates the angiogenic capability of endothelial cells. Neoplasia (New York, N.Y.) 9 (4), 349–357. doi:10.1593/neo.07133
Mitchem, J. B., Brennan, D. J., Knolhoff, B. L., Belt, B. A., Zhu, Y., Sanford, D. E., et al. (2013). Targeting tumor-infiltrating macrophages decreases tumor-initiating cells, relieves immunosuppression, and improves chemotherapeutic responses. Cancer Res. 73 (3), 1128–1141. doi:10.1158/0008-5472.CAN-12-2731
Mittelbrunn, M., and Sánchez-Madrid, F. (2012). Intercellular communication: diverse structures for exchange of genetic information. Nat. Rev. Mol. Cell Biol. 13 (5), 328–335. doi:10.1038/nrm3335
Monleón, I., Martínez-Lorenzo, M. J., Monteagudo, L., Lasierra, P., Taulés, M., Iturralde, M., et al. (2001). Differential secretion of Fas ligand- or APO2 ligand/TNF-related apoptosis-inducing ligand-carrying microvesicles during activation-induced death of human T cells. J. Immunol. 167 (12), 6736–6744. doi:10.4049/jimmunol.167.12.6736
Moris, D., Palta, M., Kim, C., Allen, P. J., Morse, M. A., and Lidsky, M. E. (2023). Advances in the treatment of intrahepatic cholangiocarcinoma: an overview of the current and future therapeutic landscape for clinicians. CA a cancer J. Clin. 73 (2), 198–222. doi:10.3322/caac.21759
Mostefai, H. A., Andriantsitohaina, R., and Martínez, M. C. (2008). Plasma membrane microparticles in angiogenesis: role in ischemic diseases and in cancer. Physiological Res. 57 (3), 311–320. doi:10.33549/physiolres.931533
Mu, W., Rana, S., and Zöller, M. (2013). Host matrix modulation by tumor exosomes promotes motility and invasiveness. Neoplasia (New York, N.Y.) 15 (8), 875–887. doi:10.1593/neo.13786
Nakagawa, H., Liyanarachchi, S., Davuluri, R. V., Auer, H., Martin, E. W., de la Chapelle, A., et al. (2004). Role of cancer-associated stromal fibroblasts in metastatic colon cancer to the liver and their expression profiles. Oncogene 23 (44), 7366–7377. doi:10.1038/sj.onc.1208013
Ota, Y., Takahashi, K., Otake, S., Tamaki, Y., Okada, M., Aso, K., et al. (2018). Extracellular vesicle-encapsulated miR-30e suppresses cholangiocarcinoma cell invasion and migration via inhibiting epithelial-mesenchymal transition. Oncotarget 9 (23), 16400–16417. doi:10.18632/oncotarget.24711
Peng, L.-S., Zhang, J.-Y., Teng, Y.-S., Zhao, Y.-L., Wang, T.-T., Mao, F.-Y., et al. (2017). Tumor-associated monocytes/macrophages impair NK-cell function via TGFβ1 in human gastric cancer. Cancer Immunol. Res. 5 (3), 248–256. doi:10.1158/2326-6066.CIR-16-0152
Poggi, A., and Giuliani, M. (2016). Mesenchymal stromal cells can regulate the immune response in the tumor microenvironment. Vaccines 4 (4), 41. doi:10.3390/vaccines4040041
Ponte, A. L., Marais, E., Gallay, N., Langonné, A., Delorme, B., Hérault, O., et al. (2007). The in vitro migration capacity of human bone marrow mesenchymal stem cells: comparison of chemokine and growth factor chemotactic activities. Stem cells Dayt. Ohio) 25 (7), 1737–1745. doi:10.1634/stemcells.2007-0054
Raggi, C., Correnti, M., Sica, A., Andersen, J. B., Cardinale, V., Alvaro, D., et al. (2017). Cholangiocarcinoma stem-like subset shapes tumor-initiating niche by educating associated macrophages. J. hepatology 66 (1), 102–115. doi:10.1016/j.jhep.2016.08.012
Rao, Q., Zuo, B., Lu, Z., Gao, X., You, A., Wu, C., et al. (2016). Tumor-derived exosomes elicit tumor suppression in murine hepatocellular carcinoma models and humans in vitro. Hepatol. Baltim. Md 64 (2), 456–472. doi:10.1002/hep.28549
Raposo, G., and Stoorvogel, W. (2013). Extracellular vesicles: exosomes, microvesicles, and friends. J. Cell Biol. 200 (4), 373–383. doi:10.1083/jcb.201211138
Ratajczak, J., Wysoczynski, M., Hayek, F., Janowska-Wieczorek, A., and Ratajczak, M. Z. (2006). Membrane-derived microvesicles: important and underappreciated mediators of cell-to-cell communication. Leukemia 20 (9), 1487–1495. doi:10.1038/sj.leu.2404296
Record, M., Carayon, K., Poirot, M., and Silvente-Poirot, S. (2014). Exosomes as new vesicular lipid transporters involved in cell-cell communication and various pathophysiologies. Biochimica Biophysica Acta 1841 (1), 108–120. doi:10.1016/j.bbalip.2013.10.004
Richards, K. E., Zeleniak, A. E., Fishel, M. L., Wu, J., Littlepage, L. E., and Hill, R. (2017). Cancer-associated fibroblast exosomes regulate survival and proliferation of pancreatic cancer cells. Oncogene 36 (13), 1770–1778. doi:10.1038/onc.2016.353
Rodewald, H.-R., and Feyerabend, T. B. (2012). Widespread immunological functions of mast cells: fact or fiction? Immunity 37 (1), 13–24. doi:10.1016/j.immuni.2012.07.007
Seo, N., Akiyoshi, K., and Shiku, H. (2018). Exosome-mediated regulation of tumor immunology. Cancer Sci. 109 (10), 2998–3004. doi:10.1111/cas.13735
Shao, H., Chung, J., Lee, K., Balaj, L., Min, C., Carter, B. S., et al. (2015). Chip-based analysis of exosomal mRNA mediating drug resistance in glioblastoma. Nat. Commun. 6, 6999. doi:10.1038/ncomms7999
Shu, L., Li, X., Liu, Z., Li, K., Shi, A., Tang, Y., et al. (2023). Bile exosomal miR-182/183-5p increases cholangiocarcinoma stemness and progression by targeting HPGD and increasing PGE2 generation. Hepatology 2023, 437. doi:10.1097/HEP.0000000000000437
Sica, A., Allavena, P., and Mantovani, A. (2008). Cancer related inflammation: the macrophage connection. Cancer Lett. 267 (2), 204–215. doi:10.1016/j.canlet.2008.03.028
Singh, R., Pochampally, R., Watabe, K., Lu, Z., and Mo, Y.-Y. (2014). Exosome-mediated transfer of miR-10b promotes cell invasion in breast cancer. Mol. cancer 13, 256. doi:10.1186/1476-4598-13-256
Sirica, A. E., Almenara, J. A., and Li, C. (2014). Periostin in intrahepatic cholangiocarcinoma: pathobiological insights and clinical implications. Exp. Mol. pathology 97 (3), 515–524. doi:10.1016/j.yexmp.2014.10.007
Sirica, A. E., Gores, G. J., Groopman, J. D., Selaru, F. M., Strazzabosco, M., Wei Wang, X., et al. (2019). Intrahepatic cholangiocarcinoma: continuing challenges and translational advances. Hepatol. Baltim. Md 69 (4), 1803–1815. doi:10.1002/hep.30289
Skokos, D., Botros, H. G., Demeure, C., Morin, J., Peronet, R., Birkenmeier, G., et al. (2003). Mast cell-derived exosomes induce phenotypic and functional maturation of dendritic cells and elicit specific immune responses in vivo. J. Immunol. 170 (6), 3037–3045. doi:10.4049/jimmunol.170.6.3037
Spaeth, E., Klopp, A., Dembinski, J., Andreeff, M., and Marini, F. (2008). Inflammation and tumor microenvironments: defining the migratory itinerary of mesenchymal stem cells. Gene Ther. 15 (10), 730–738. doi:10.1038/gt.2008.39
Spaeth, E. L., Dembinski, J. L., Sasser, A. K., Watson, K., Klopp, A., Hall, B., et al. (2009). Mesenchymal stem cell transition to tumor-associated fibroblasts contributes to fibrovascular network expansion and tumor progression. PloS One 4 (4), e4992. doi:10.1371/journal.pone.0004992
Stacker, S. A., Caesar, C., Baldwin, M. E., Thornton, G. E., Williams, R. A., Prevo, R., et al. (2001). VEGF-D promotes the metastatic spread of tumor cells via the lymphatics. Nat. Med. 7 (2), 186–191. doi:10.1038/84635
Subra, C., Grand, D., Laulagnier, K., Stella, A., Lambeau, G., Paillasse, M., et al. (2010). Exosomes account for vesicle-mediated transcellular transport of activatable phospholipases and prostaglandins. J. lipid Res. 51 (8), 2105–2120. doi:10.1194/jlr.M003657
Subra, C., Laulagnier, K., Perret, B., and Record, M. (2007). Exosome lipidomics unravels lipid sorting at the level of multivesicular bodies. Biochimie 89 (2), 205–212. doi:10.1016/j.biochi.2006.10.014
Takagi, S., Miyagawa, S.-I., Ichikawa, E., Soeda, J., Miwa, S., Miyagawa, Y., et al. (2004). Dendritic cells, T-cell infiltration, and Grp94 expression in cholangiocellular carcinoma. Hum. Pathol. 35 (7), 881–886. doi:10.1016/j.humpath.2004.03.016
Taraboletti, G., D'Ascenzo, S., Giusti, I., Marchetti, D., Borsotti, P., Millimaggi, D., et al. (2006). Bioavailability of VEGF in tumor-shed vesicles depends on vesicle burst induced by acidic pH. Neoplasia (New York, N.Y.) 8 (2), 96–103. doi:10.1593/neo.05583
Techasen, A., Loilome, W., Namwat, N., Dokduang, H., Jongthawin, J., and Yongvanit, P. (2012). Cytokines released from activated human macrophages induce epithelial mesenchymal transition markers of cholangiocarcinoma cells. Asian Pac. J. cancer Prev. APJCP 13 (1), 115–118.
Thakur, B. K., Zhang, H., Becker, A., Matei, I., Huang, Y., Costa-Silva, B., et al. (2014). Double-stranded DNA in exosomes: a novel biomarker in cancer detection. Cell Res. 24 (6), 766–769. doi:10.1038/cr.2014.44
Thanee, M., Loilome, W., Techasen, A., Namwat, N., Boonmars, T., Pairojkul, C., et al. (2015). Quantitative changes in tumor-associated M2 macrophages characterize cholangiocarcinoma and their association with metastasis. Asian Pac. J. cancer Prev. APJCP 16 (7), 3043–3050. doi:10.7314/apjcp.2015.16.7.3043
Tkach, M., Thalmensi, J., Timperi, E., Gueguen, P., Névo, N., Grisard, E., et al. (2022). Extracellular vesicles from triple negative breast cancer promote pro-inflammatory macrophages associated with better clinical outcome. Proc. Natl. Acad. Sci. U. S. A. 119 (17), e2107394119. doi:10.1073/pnas.2107394119
Toshida, K., Itoh, S., Harada, N., Morinaga, A., Yugawa, K., Tomiyama, T., et al. (2023). Cancer-associated fibroblasts promote tumor cell growth via miR-493-5p in intrahepatic cholangiocarcinoma. Cancer Sci. 114 (3), 937–947. doi:10.1111/cas.15644
Trajkovic, K., Hsu, C., Chiantia, S., Rajendran, L., Wenzel, D., Wieland, F., et al. (2008). Ceramide triggers budding of exosome vesicles into multivesicular endosomes. Sci. (New York, N.Y.) 319 (5867), 1244–1247. doi:10.1126/science.1153124
Tran, E., Turcotte, S., Gros, A., Robbins, P. F., Lu, Y.-C., Dudley, M. E., et al. (2014). Cancer immunotherapy based on mutation-specific CD4+ T cells in a patient with epithelial cancer. Science 344 (6184), 641–645. doi:10.1126/science.1251102
Trifylli, E.-M., Kriebardis, A. G., Koustas, E., Papadopoulos, N., Vasileiadi, S., Fortis, S. P., et al. (2023). The arising role of extracellular vesicles in cholangiocarcinoma: a rundown of the current knowledge regarding diagnostic and therapeutic approaches. Int. J. Mol. Sci. 24 (21), 15563. doi:10.3390/ijms242115563
Tsukagoshi, M., Wada, S., Yokobori, T., Altan, B., Ishii, N., Watanabe, A., et al. (2016). Overexpression of natural killer group 2 member D ligands predicts favorable prognosis in cholangiocarcinoma. Cancer Sci. 107 (2), 116–122. doi:10.1111/cas.12853
Vaeteewoottacharn, K., Kariya, R., Dana, P., Fujikawa, S., Matsuda, K., Ohkuma, K., et al. (2016). Inhibition of carbonic anhydrase potentiates bevacizumab treatment in cholangiocarcinoma. Tumour Biol. J. Int. Soc. Oncodevelopmental Biol. Med. 37 (7), 9023–9035. doi:10.1007/s13277-016-4785-8
Vaquero, J., Aoudjehane, L., and Fouassier, L. (2020). Cancer-associated fibroblasts in cholangiocarcinoma. Curr. Opin. gastroenterology 36 (2), 63–69. doi:10.1097/MOG.0000000000000609
Wadsworth, C. A., Dixon, P. H., Taylor-Robinson, S., Kim, J. U., Zabron, A. A., Wong, J. H., et al. (2019). Polymorphisms in natural killer cell receptor protein 2D (NKG2D) as a risk factor for cholangiocarcinoma. J. Clin. Exp. hepatology 9 (2), 171–175. doi:10.1016/j.jceh.2018.06.521
Wahlgren, J., Karlson, T. D. L., Glader, P., Telemo, E., and Valadi, H. (2012). Activated human T cells secrete exosomes that participate in IL-2 mediated immune response signaling. PloS one 7 (11), e49723. doi:10.1371/journal.pone.0049723
Waldenström, A., Gennebäck, N., Hellman, U., and Ronquist, G. (2012). Cardiomyocyte microvesicles contain DNA/RNA and convey biological messages to target cells. PloS one 7 (4), e34653. doi:10.1371/journal.pone.0034653
Wang, J., and Ilyas, S. (2021). Targeting the tumor microenvironment in cholangiocarcinoma: implications for therapy. Expert Opin. investigational drugs 30 (4), 429–438. doi:10.1080/13543784.2021.1865308
Wang, S., Sun, J., Dastgheyb, R. M., and Li, Z. (2022). Tumor-derived extracellular vesicles modulate innate immune responses to affect tumor progression. Front. Immunol. 13, 1045624. doi:10.3389/fimmu.2022.1045624
Wang, X., Li, X., Wei, X., Jiang, H., Lan, C., Yang, S., et al. (2020). PD-L1 is a direct target of cancer-FOXP3 in pancreatic ductal adenocarcinoma (PDAC), and combined immunotherapy with antibodies against PD-L1 and CCL5 is effective in the treatment of PDAC. Signal Transduct. Target. Ther. 5 (1), 38. doi:10.1038/s41392-020-0144-8
Xie, F., Feng, S., Yang, H., and Mao, Y. (2019). Extracellular vesicles in hepatocellular cancer and cholangiocarcinoma. Ann. Transl. Med. 7 (5), 86. doi:10.21037/atm.2019.01.12
Xie, Y., Zhang, H., Li, W., Deng, Y., Munegowda, M. A., Chibbar, R., et al. (2010). Dendritic cells recruit T cell exosomes via exosomal LFA-1 leading to inhibition of CD8+ CTL responses through downregulation of peptide/MHC class I and Fas ligand-mediated cytotoxicity. J. Immunol. 185 (9), 5268–5278. doi:10.4049/jimmunol.1000386
Xu, Y., Leng, K., Yao, Y., Kang, P., Liao, G., Han, Y., et al. (2021). A circular RNA, cholangiocarcinoma-associated circular RNA 1, contributes to cholangiocarcinoma progression, induces angiogenesis, and Disrupts vascular endothelial barriers. Hepatol. Baltim. Md 73 (4), 1419–1435. doi:10.1002/hep.31493
Yamada, D., Rizvi, S., Razumilava, N., Bronk, S. F., Davila, J. I., Champion, M. D., et al. (2015). IL-33 facilitates oncogene-induced cholangiocarcinoma in mice by an interleukin-6-sensitive mechanism. Hepatol. Baltim. Md 61 (5), 1627–1642. doi:10.1002/hep.27687
Yamamoto, Y., Sugimoto, A., Maruo, K., Tsujio, G., Sera, T., Kushiyama, S., et al. (2022). CXCR2 signaling might have a tumor-suppressive role in patients with cholangiocarcinoma. PloS one 17 (4), e0266027. doi:10.1371/journal.pone.0266027
Yáñez-Mó, M., Siljander, P. R. M., Andreu, Z., Zavec, A. B., Borràs, F. E., Buzas, E. I., et al. (2015). Biological properties of extracellular vesicles and their physiological functions. J. Extracell. vesicles 4, 27066. doi:10.3402/jev.v4.27066
Yang, F., Ning, Z., Ma, L., Liu, W., Shao, C., Shu, Y., et al. (2017). Exosomal miRNAs and miRNA dysregulation in cancer-associated fibroblasts. Mol. cancer 16 (1), 148. doi:10.1186/s12943-017-0718-4
Yang, X., Lin, Y., Shi, Y., Li, B., Liu, W., Yin, W., et al. (2016). FAP promotes immunosuppression by cancer-associated fibroblasts in the tumor microenvironment via STAT3-CCL2 signaling. Cancer Res. 76 (14), 4124–4135. doi:10.1158/0008-5472.CAN-15-2973
Yang, Y., Bucan, V., Baehre, H., von der Ohe, J., Otte, A., and Hass, R. (2015). Acquisition of new tumor cell properties by MSC-derived exosomes. Int. J. Oncol. 47 (1), 244–252. doi:10.3892/ijo.2015.3001
Yeh, C.-N., Chang, Y.-C., Su, Y., Shin-Shian Hsu, D., Cheng, C.-T., Wu, R.-C., et al. (2017). Identification of MALT1 as both a prognostic factor and a potential therapeutic target of regorafenib in cholangiocarcinoma patients. Oncotarget 8 (69), 113444–113459. doi:10.18632/oncotarget.23049
Yuan, H., Lin, Z., Liu, Y., Jiang, Y., Liu, K., Tu, M., et al. (2020). Intrahepatic cholangiocarcinoma induced M2-polarized tumor-associated macrophages facilitate tumor growth and invasiveness. Cancer Cell Int. 20 (1), 586. doi:10.1186/s12935-020-01687-w
Zanotti, L., Sarukhan, A., Dander, E., Castor, M., Cibella, J., Soldani, C., et al. (2013). Encapsulated mesenchymal stem cells for in vivo immunomodulation. Leukemia 27 (2), 500–503. doi:10.1038/leu.2012.202
Zhang, B., Yin, Y., Lai, R. C., Tan, S. S., Choo, A. B. H., and Lim, S. K. (2014). Mesenchymal stem cells secrete immunologically active exosomes. Stem cells Dev. 23 (11), 1233–1244. doi:10.1089/scd.2013.0479
Zhang, H.-G., and Grizzle, W. E. (2011). Exosomes and cancer: a newly described pathway of immune suppression. Clin. cancer Res. official J. Am. Assoc. Cancer Res. 17 (5), 959–964. doi:10.1158/1078-0432.CCR-10-1489
Zhang, M., Yang, H., Wan, L., Wang, Z., Wang, H., Ge, C., et al. (2020). Single-cell transcriptomic architecture and intercellular crosstalk of human intrahepatic cholangiocarcinoma. J. hepatology 73 (5), 1118–1130. doi:10.1016/j.jhep.2020.05.039
Zhang, X.-F., Dong, M., Pan, Y.-H., Chen, J.-N., Huang, X.-Q., Jin, Y., et al. (2017). Expression pattern of cancer-associated fibroblast and its clinical relevance in intrahepatic cholangiocarcinoma. Hum. Pathol. 65, 92–100. doi:10.1016/j.humpath.2017.04.014
Zheng, X., Xu, M., Yao, B., Wang, C., Jia, Y., and Liu, Q. (2016). IL-6/STAT3 axis initiated CAFs via up-regulating TIMP-1 which was attenuated by acetylation of STAT3 induced by PCAF in HCC microenvironment. Cell. Signal. 28 (9), 1314–1324. doi:10.1016/j.cellsig.2016.06.009
Zhou, Z., Wang, P., Sun, R., Li, J., Hu, Z., Xin, H., et al. (2021). Tumor-associated neutrophils and macrophages interaction contributes to intrahepatic cholangiocarcinoma progression by activating STAT3. J. Immunother. cancer 9 (3), e001946. doi:10.1136/jitc-2020-001946
Keywords: cholangiocarcinoma, tumor microenvironment, extracellular vesicles, exosome, EV-based therapy
Citation: Zhang N, Shu L, Liu Z, Shi A, Zhao L, Huang S, Sheng G, Yan Z, Song Y, Huang F, Tang Y and Zhang Z (2024) The role of extracellular vesicles in cholangiocarcinoma tumor microenvironment. Front. Pharmacol. 14:1336685. doi: 10.3389/fphar.2023.1336685
Received: 11 November 2023; Accepted: 26 December 2023;
Published: 10 January 2024.
Edited by:
Jiajie Peng, Northwestern Polytechnical University, ChinaReviewed by:
Hongbo Xu, Central South University, ChinaRamcharan Singh Angom, Mayo Clinic Florida, United States
Copyright © 2024 Zhang, Shu, Liu, Shi, Zhao, Huang, Sheng, Yan, Song, Huang, Tang and Zhang. This is an open-access article distributed under the terms of the Creative Commons Attribution License (CC BY). The use, distribution or reproduction in other forums is permitted, provided the original author(s) and the copyright owner(s) are credited and that the original publication in this journal is cited, in accordance with accepted academic practice. No use, distribution or reproduction is permitted which does not comply with these terms.
*Correspondence: Zongli Zhang, zzlzzl1900@163.com; Yongchang Tang, tangyongchang@sdu.edu.cn
†These authors have contributed equally to this work and share first authorship