- Institute of Cell Biology (Cancer Research), Medical Faculty, University of Duisburg–Essen, Essen, Germany
A hallmark of T cell dependent (TD) humoral immune responses is the generation of long–lived memory B cells. The generation of these cells occurs primarily in the germinal center (GC) reaction, where antigen–activated B cells undergo affinity maturation as a major consequence of the combined processes of proliferation, somatic hypermutation of their immunoglobulin V (IgV) region genes, and selection for improved affinity of their B–cell antigen receptors. As many B cells also undergo class–switching to IgG or IgA in these TD responses, there was traditionally a focus on class–switched memory B cells in both murine and human studies on memory B cells. However, it has become clear that there is also a large subset of IgM–expressing memory B cells, which have important phenotypic and functional similarities but also differences to class–switched memory B cells. There is an ongoing discussion about the origin of distinct subsets of human IgM+ B cells with somatically mutated IgV genes. We argue here that the vast majority of human IgM–expressing B cells with somatically mutated IgV genes in adults is indeed derived from GC reactions, even though a generation of some mostly lowly mutated IgM+ B cells from other differentiation pathways, mainly in early life, may exist.
Introduction
A major strength of the human immune system is that it is not only capable of combating infectious agents, such as viruses and bacteria, during a first encounter with these pathogenic microorganisms, but that it also establishes immunological memory. This immunological memory prepares our immune system for a faster and improved response upon re–encounter with the same or a related infectious agent. Immunological memory is a feature of the lymphocytes of the adaptive immune system, i.e., B cells and T cells. B lymphocytes recognize foreign antigens through their B–cell antigen receptor (BCR), which can be secreted as soluble antibody if B cells differentiate into plasmablasts or plasma cells. Memory within the B–cell system is largely, if not exclusively, generated during T cell dependent (TD) immune responses, which include the germinal center (GC) reaction. In the GC reaction, two types of long–lived descendants of antigen–activated B cells are generated, namely memory B cells and plasma cells (1). Whereas plasma cells have no further proliferative potential and cannot modify the fine–specificity of the antibody molecules they produce, memory B cells are more flexible in their behavior upon re–encounter of the antigen for which their BCR is specific. After reactivation they can either proliferate and then become plasma cells, or they may reenter a GC reaction for further improvement of the affinity of their BCR or its adaptation to a modified antigen (2). Distinct subsets of human memory B cells have been identified, and a major distinction is between IgM–expressing and class–switched memory B cells (2). However, there is still an ongoing discussion about the origin, heterogeneity, and specific functions of IgM–expressing memory B cells in humans. This review is focused on discussing the current knowledge and ideas about human IgM+ memory B cells.
B–cell development
Human B lymphocytes are generated from common lymphoid progenitors in a stepwise differentiation process that takes place in postnatal life in the bone marrow (3). The main task of early B–cell development is to equip each B cell with a BCR. This is mediated through several somatic recombination events which assemble the variable parts of the immunoglobulin (Ig) heavy and light chain genes, mediated by the enzymes recombination activating gene (RAG)1 and RAG2 (4). For the heavy chain, three gene segments have to be assembled, the variable (V), diversity (D), and joining (J) genes, whereas the kappa and lambda light chain V region genes are composed of a V and a J gene (5). The estimated potential diversity of the human mature BCR repertoire is 1012 to 1018 unique rearrangements (6). Several factors contribute to this enormous diversity of the BCR repertoire: First, there is a substantial number of different germline IGHV, IGHD and IGHJ genes that can be recombined to encode the Ig heavy chain V region, and numerous IgV and IgJ genes can be assembled to generate the kappa and lambda light chain V regions in a process called V(D)J–recombination (5, 7). Second, the random integration and removal of nucleotides at the junctions of the rearranging genes massively increases diversity (7). Third, multiple combinations of rearranged Ig heavy and light chains can pair to form a stable BCR. These mechanisms lead to the generation of naive, mature B cells, which are equipped with a unique BCR. The unique nucleotide sequence [in particular that of the complementarity determining region (CDR) III of the heavy chain, which encompasses the IGHV–IGHD–IGHJ joining site] of the BCR can be used as a clonal marker to trace the progeny of this single cell during immune responses. Mature B cells exit the bone marrow and circulate through the body in search for foreign antigens. Naive B cells coexpress the BCR as IgM and IgD molecules, which is mediated through differential splicing of the primary heavy chain transcripts. These cells can be identified in flow cytometric studies through staining with a combination of antibodies (see Figure 1).
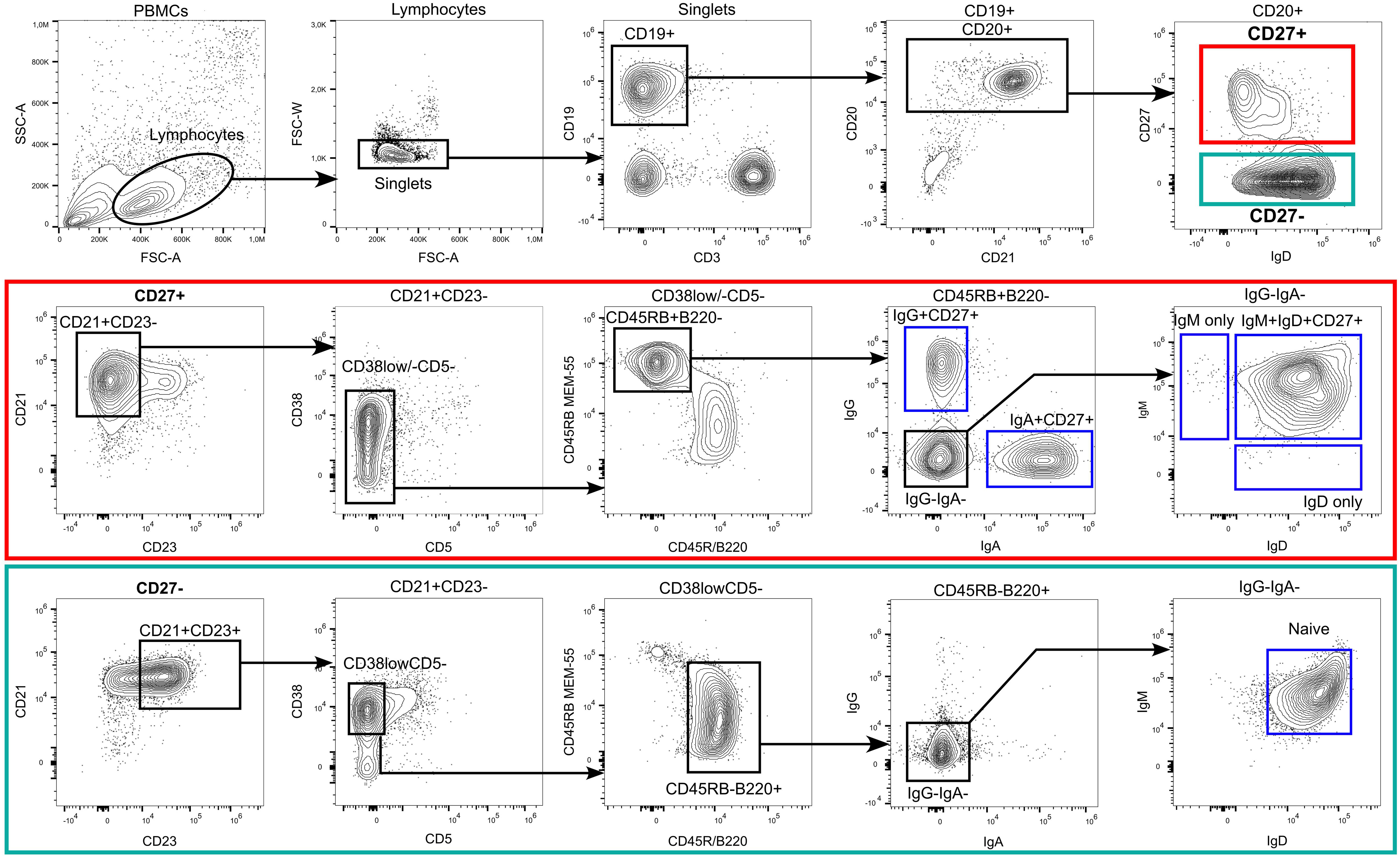
Figure 1 Flow cytometric identification of human B–cell subsets. Among peripheral blood–derived mononuclear cells (PBMC), most mature B cells can be identified by the expression of the pan B cell markers CD19 and/or CD20. CD19 also stains plasma blasts and most plasma cells, which are mostly CD20lowCD21low and surface BCRlow in peripheral blood. The choice of one or more of these molecules, to include into the FACS panel depends on the B–cell subsets of interest, expression of additional B–cell hallmark molecules, such as the heavy or light chains of the BCR, and the configuration of the flow cytometer. Exclusion of non–B–cells is often recommendable, as is depicted in the plot for the CD19 and CD3 staining. In addition, markers such as CD56 (natural killer cells), CD14, and CD16 (monocytes and granulocytes) can be co–stained using the same fluorescent dye as CD3 (creating a dump channel; not shown here). Mature naive and memory B cells can be distinguished by the differential expression of CD23, CD27, CD45RB (clone MEM–55), CD45R/B220 (clone RA3–6B2) and the expression of class–switched BCR isotypes such as IgG and IgA. Mature naive B cells express the phenotype CD23posCD27negCD45RBnegB220posCD38lowCD5negIgGnegIgAnegIgDposIgMpos. However, not all of these markers are mandatory for a routine identification of naive B cells, for which we recommend stainings at least for CD19 or CD20, IgD, CD23, and CD27. Most memory B cells express the phenotype CD23negCD27posCD45RBposB220neg and can be distinguished by their expression of surface BCR isotypes (IgM/IgG/IgA/IgE). In addition, a small fraction of CD27negIgApos and CD27negIgGpos memory B cells exist in human blood and lymphoid tissues. A core panel to define class–switched memory B cells should include CD19 or CD20, IgG and/or IgA, and CD27. Please note that the frequently used marker constellation IgD–CD27+ also includes a small subset of IgM–only memory B cells. In addition, small subsets of CD27negIgApos and CD27negIgGpos memory B cells exist in human blood and lymphoid tissues. CD24 and CD10 can support the differentiation of mature B cells from transitional or immature B cells (transitional B cells express CD24highCD10high in addition to CD38posCD5pos), which is particularly important when analyzing bone marrow, and from GC B cells (CD24lowCD10high in addition to CD38pos), which is of interest when analyzing secondary lymphoid organs. Additional subsets that were not pointed out in the gating strategy include atypical B cells (CD21lowTbetposCD183posCD11cpos) and CD5 mature naive B cells (CD27negCD23posCD38lowCD5pos). The CD5 mature B cells in adults are phenotypically and functionally similar to CD5neg naive B cells, however, in neonates and children these two populations differ substantially (8).
Germinal center reaction
TD immune responses are initiated when antigen–activated B cells meet antigen–specific T helper (TH) cells in the T–cell region or the T–B region border of secondary lymphoid organs, such as lymph nodes. Antigen– and TH cell–stimulated B cells then proliferate, building a primary focus (9). Some of the B cells differentiate into short lived plasma cells, and studies in the mouse showed that they can also differentiate into TD memory B cells with unmutated IgV genes without participating in the GC reaction (10). Other B cells of the primary focus reaction migrate into neighboring B–cell follicles. In the follicle, the activated B cells undergo massive proliferation, thereby establishing a histological structure called GC (1). The GC reaction is the major process that generates memory B cells with high–affinity BCRs and plasma cells secreting high affinity antibodies in a TD fashion. The main players besides the antigen–activated GC B cells are T follicular helper (TFH) cells and follicular dendritic cells (FDCs). B cells in the GC undergo regulated cycles of proliferation, IgV gene mutation, and selection for improved affinity of their BCR. A GC can be histologically subdivided into the dark zone and the light zone (1). GC B cells in the dark zone (centroblasts) vigorously proliferate and undergo somatic hypermutation (SHM) of their Ig heavy and light chain V region genes (11, 12). SHM is initiated by activation–induced cytidine deaminase (AID), which deaminates C bases, giving rise to U bases (13). Error–prone repair of the U bases then leads to the generation of somatic mutations. After a few cell divisions, centroblasts cease proliferation and SHM and move to the light zone of the GC where they become resting centrocytes (1). Centrocytes are then selected for improved binding of their BCR to the cognate antigen, which involves presentation of antigenic peptides loaded on major histocompatibility complex II molecules to the T–cell receptor of TFH cells (1, 14). The selection and differentiation process of GC B cells involves further receptor–ligand interactions, such as binding of CD40 on GC B cells to CD40L on TFH cells, and secreted factors, such as interleukins 4 and 21 (1). The vast majority of GC B cells will not acquire affinity–increasing mutations, and these cells rapidly undergo apoptosis (1). GC B cells typically undergo multiple rounds of proliferation, SHM and selection, leading to a stepwise improvement of BCR affinity. TFH cells also direct the further differentiation of GC B cells into either memory B cells or plasma cells (1). Memory B cells are often generated earlier in a GC reaction than plasma cells, as the antibodies produced by plasma cells often have a higher somatic mutation load and a higher affinity for the antigen than the BCR of memory B cells (15).
A further Ig gene remodeling process is class switch recombination (CSR). This process is, like SHM, mediated by AID, which causes DNA double strand breaks in the switch regions upstream of the IGH constant region genes (13). CSR leads to the exchange of the constant part of the Ig heavy chain, so that instead of co–expression of IgM and IgD now immunoglobulins of the classes IgG, IgA and IgE with distinct effector functions can be produced. Whereas initial findings point to a predominant activity of CSR in the light zone of the GC, recent findings in mice and humans indicate that many antigen-activated B cells in TD immune responses undergo CSR already prior to entering the GC (2, 16, 17).
A cell surface receptor with high relevance for the further discussion is the tumor necrosis factor receptor (TNFR) superfamily member CD27. CD27 is not expressed by naive B cells, is upregulated on GC B cells and remains expressed on most memory B cells (18–20). It is highly expressed on post–GC plasma cells (20, 21). CD27 has been proposed as a general memory B–cell marker in humans (18, 19).
Human memory B–cell subsets
Historically, memory B cells were identified by the expression of class–switched BCR, in particular IgG and IgA. This is because CSR was considered as a main hallmark of B cells that underwent a GC reaction and became memory B cells, and as surface IgG and IgA can be easily used to detect and isolate these cells. However, it is now clear that also IgM–expressing memory B cells exist, and further subsets of memory B cells have been identified (Figure 2). Before discussing IgM–expressing memory B cells – the main topic of this review – we provide a short overview over the other main subsets of human memory B cells.
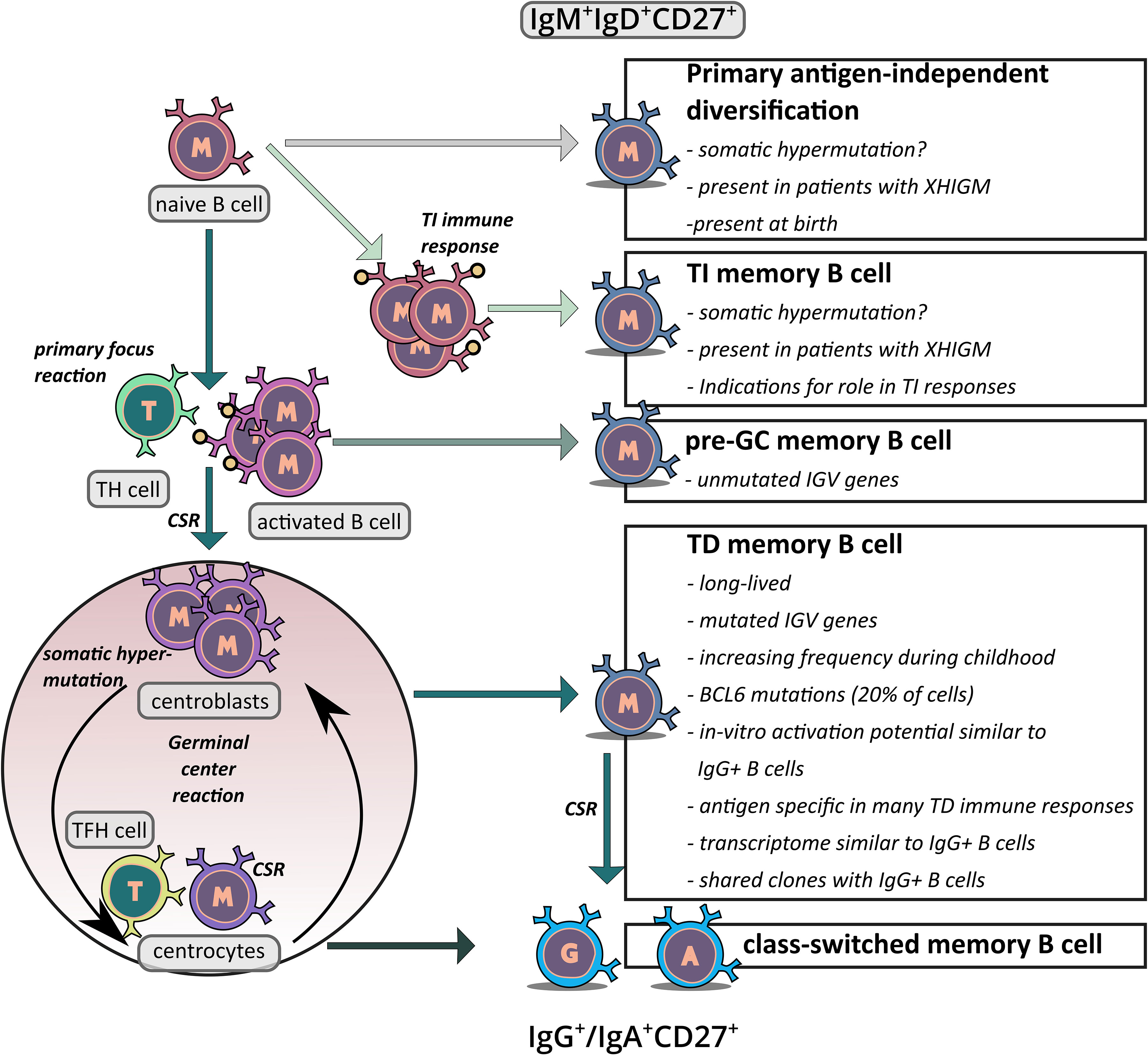
Figure 2 Schematic overview of the proposed generation pathways of IgM+IgD+CD27+ memory B cells. The figure depicts the potential origins of IgM+IgD+CD27+ B cells. First, there is indication that at least in young children, a subset of these cells may be derived from an antigen–independent primary diversification process in which the cells acquire a low level of somatic mutations. Second, it has been proposed that IgM+IgD+CD27+ B cells are derived from TI immune responses and hence represent memory B cells which were generated without T cell help. Third, in TD immune responses some memory B cells may be generated in the primary focus reaction when cognate B and T cells have their first interaction, before SHM is activated. This could be the origin of IgM+IgD+CD27+ B cells without IGV gene mutations. Fourth, at least in adults the vast majority of IgM+IgD+CD27+ B cells presumably derives from TD GC responses, which also give rise the classical class–switched memory B cells. Key arguments for and unresolved issues of the differentiation pathways discussed are indicated in the boxes.
IgG memory B cells
In humans, there are four functional IgG isotypes (IgG1, IgG2, IgG3, and IgG4). About 80% of IgG memory B cells express the CD27 marker, and IgG+ B cells typically account for about 10–15% of B cells in the peripheral blood of healthy adults (19, 22–24) (Figure 1, Table 1). IgG1 and IgG2 are most frequently used by IgG memory B cells, whereas IgG4+ B cells are very rare (24–26). Nearly all IgG+ memory B cells carry somatically mutated IgV genes, with average mutation frequencies of 5–7% (19, 25, 27, 28). As a hallmark of memory B cells, IgG+ memory B cells can be faster and stronger activated than naive B cells (29–37). In vitro, such reactivated IgG memory B cells have a propensity to differentiate into plasmablasts and plasma cells, instead of acquiring a GC program (29).
IgA memory B cells
IgA+ memory B cells account for about 10% of B cells in the peripheral blood of healthy adults (24). Similar to IgG memory B cells they mostly express CD27, and the vast majority of CD27+IgA+ memory B cells carry somatically mutated IgV genes, at levels similar to IgG memory B cells (28, 38). IgA+ memory B cells are mostly generated in the intestine and other mucosal tissues. The most important IgA–inductive sites in the gut are Peyer’s patches (PP), clusters of follicles where IgA+ B cells are generated (39, 40). The second structure responsible for IgA B–cell development are isolated lymphoid follicles, which are smaller than PPs and often contain only one follicle (39). In mice there are comprehensive data that a fraction of IgA memory B cells and plasma cells are generated in T–cell independent (TI) immune responses (41, 42). However, in humans the high level of somatic IgV gene mutations seen in the vast majority of IgA+CD27+ memory B cells and plasma cells argues that these cells are mainly generated in GC reactions of TD immune responses (38, 43, 44).
IgD–only B cells
A very rare (<1% of B cells in peripheral blood) and peculiar subset of memory B cells is characterized by expression of IgD in the complete absence of IgM, so–called IgD–only B cells (19, 45). Whereas mature naive B cells and IgM–expressing memory B cells co–express IgM and IgD, and may finetune their relative expression depending on the particular differentiation and activation stage (46), IgD–only B cells have undergone an atypical CSR event involving the Sμ region and a pentamer–rich σ/δ region upstream of Cδ (47), causing deletion of all Cμ exons, so that these cells cannot express any IgM anymore. IgD–only B cells were first identified as the malignant clones in some patients with multiple myeloma (48) and hairy cell leukemia (49). IgD–only memory B cells derive from IgD–only GC B cells, which are mainly found in tonsils, and these GC B cells also give rise to IgD–secreting plasma cells (47, 50). IgD–only B cells show a very high IgV gene mutation frequency, a highly biased usage of λ light chains, and a preferred usage of the IGHV3–30 gene (19, 50, 51). These features indicate that those cells derive from a peculiar, likely super–antigen–driven, GC reaction (19, 50, 51).
IgE memory B cells
IgE+ memory B cells are very rare in the human body and account for about 0.1% of peripheral blood B cells in adults (52). IgE has a physiological role in immune responses against parasites, but it has also a main role in allergic reactions, as IgE antibodies are capable of activating mast cells (53). In mice, IgE+ GC B cells are produced early during the immune response and then quickly disappear (54–56). It is not clear if this is also the case in humans, but the current concept in mice and humans is that the main source of IgE–producing plasma cells is not the very small pool of IgE+ memory B cells, but IgG memory B cells that upon particular stimuli undergo secondary CSR to IgE and then differentiate into IgE–secreting plasma cells (55, 57).
CD27–negative memory B cells
As already briefly mentioned, CD27, a cell surface receptor contributing to B–cell differentiation, expansion, antibody production, and B cell–T cell–interaction (via its ligand CD70) is often used to distinguish memory B cells from naive B cells, as naive B cells lack CD27 expression and most memory B cells express CD27 on their surface (18, 19, 58, 59). CD27+ memory B cells are IgV gene mutated and often found as classical post–GC class–switched B cells (60, 61). But not all cells without CD27 are naive B cells. CD27–negative memory B cells (recognized as such because of their mutated IgV genes) represent about 5% of all B cells in the periphery in healthy adults and are already present at low frequency at birth (22, 62–64). It was proposed that such CD27– and also CD27dull memory B cells may be a form of precursor for CD27+ memory B cells (65), as there exists a high degree of similarity between the BCR repertoires of CD27– and CD27+ memory B cells, and they are frequently members of common clones (28). Moreover, IgG+CD27– memory B cells express a lower level of somatic IgV gene mutations compared to their CD27–positive counterpart (22) and single cell targeted DNA methylation analysis showed a progress of cellular differentiation with increase of CD27 expression (66), which may further support this theory.
CD21lowT–bethigh B cells
Typically, all mature B cells express the complement receptor 2 (CR2, also termed CD21). A distinct subset of CD21low mature B cells was first described in immune deficiency conditions, such as in patients with HIV infection (67, 68), but were later also identified in other chronic viral and parasitic infections, and in patients with autoimmune diseases (69, 70). In healthy individuals, these cells are very rare in peripheral blood. A corresponding population has been found at increased frequency in old mice and were hence termed age–associated B cells in these animals (71). Most of the CD21low B cells express the transcription factor T–bet at high level, which was originally described as a master regulator of commitment of CD4+ T cells to the T helper 1 (TH1) cell lineage (72). The CD21lowT–bethigh B cells are often also positive for CD11c and FCRL4 (69, 70). As different combinations of these markers were used in the various studies of CD21low and/or T–bethigh B cells, which are often also called atypical B cells, it is not clear whether this is a homogenous B–cell subset or whether in distinct disease settings different subtypes of these cells with distinct marker combinations exist. Indeed, in the various diseases, the proportions of CD27+ and class–switched B cells among these cells varies considerably (69, 70). Importantly, however, CD21lowT–bethigh B cells show strong indications of being memory B cells. Most of these cells carry somatically mutated IgV genes at levels similar to classical human memory B cells, they need T–cell help for their generation, they express pathogen–specific BCRs in chronic infections, they are often CD27– and/or IgG–expressing, and they can include common clones with classical CD27+CD21+ memory B cells (69, 70, 73, 74). Initially, CD21lowT–bethigh B cells were considered as exhausted B cells, as they responded poorly to BCR cross–linking. However, later studies showed that if BCR triggering is combined with other stimuli, e.g., specific cytokines and/or Toll–like receptor triggering, these cells responded similarly fast and strong as classical memory B cells (69, 70).
Potential origins of somatically mutated human IgM+ B cells
It is well established that most if not all class–switched memory B cells in humans are generated during GC reactions in TD immune responses. However, the generation of IgM+IgD+ B cells with somatically mutated IgV genes is still debated. Three different possible origins of these cells were proposed: the “classical” GC–derived pathway, an antigen independent primary diversification developmental pathway, and a derivation from TI immune responses (61) (Figure 2). The possible GC derivation of these cells will be discussed in detail in the following chapter. Here, we address the two alternative developmental pathways for the generation of somatically mutated IgM+IgD+ B cells.
The first indication for a GC–independent origin of human somatically mutated IgM+IgD+CD27+ B cells derived from the observation that such cells can be identified in patients affected by the X–linked hyper–IgM syndrome type 1 (HIGM1) (75). In these patients the CD40L gene is defective, which is thought to be essential for GC reactions, and class–switched memory B cells are indeed absent in these patients (75). As IgM+IgD+CD27+ B cells appear in young children before class–switched memory B cells are detectable, and develop early on a diversified BCR repertoire, this may indicate a GC and even immune response independent generation of these cells (76). A primary, antigen–independent BCR diversification through SHM is indeed known from some animal species (77). In further support of their antigen–independent generation, IgM+IgD+CD27+ B cells were also identified during human fetal development, where one does not expect immune responses to take place (78). Importantly, however, in these early life and disease settings, only a (small) fraction of the IgM+IgD+CD27+ B cells carried somatic IgV gene mutations, and the mutation frequencies in the mutated cells were mostly low (75, 76, 78). Thus, these cells did not show the typical mutation features of adolescent and adult IgM+IgD+CD27+ B cells. Moreover, it seems that there is not a complete absence of GC–like structures in HIGM1 patients (79), and a potential alternative CD40 receptor has been identified (80), so that a GC derivation of the IgM+IgD+CD27+ B cells is still possible.
Regarding an antigen–independent SHM during early B–cell development, it has also been reported that human transitional B cells (a developmental stage between immature B cells in the bone marrow and mature naive B cells) express low levels of AID (81), and that SHM can be induced in vitro in such B cells through toll–like receptor 9 (TLR9) ligation (82). However, the very low levels of mutations showed a highly untypical pattern, with an accumulation mainly in the framework regions of the V region genes and a restriction to some IGHV subgroups (82). Thus, it is highly unlikely that this is the derivation of the mutations in IgM+IgD+CD27+ B cells. Moreover, there is indication that those immature B cells that express AID are destined to undergo apoptosis (83), further arguing against the idea that this is a pathway to establish a major pool of B cells with mutated IgV genes.
A second scenario for the origin of somatically mutated IgM+IgD+CD27+ B cells is their generation and SHM in TI immune responses. This idea was originally based on the observation that splenectomized and asplenic patients show a specific strong reduction of IgM+IgD+CD27+ B cells, and a predisposition to pneumococcal infections as well as impaired responses to polysaccharide vaccines, which are both thought to be mainly mediated by TI immune responses (61, 84). This led to the concept that IgM+IgD+CD27+ B cells are generated and undergo SHM in TI immune responses, and hence represent TI memory B cells (84). However, according to other studies, removal of the spleen also substantially diminishes frequencies of class–switched memory B cells in the peripheral blood (85–88). It is also an unresolved issue where such TI immune responses accompanied by accumulation of substantial loads of somatic mutations in IgV genes take place (see Box 1). A further major caveat in the interpretation of immune responses to TI antigens in humans relates to their potential immune history. In a detailed study of the humoral immune response to a polysaccharide vaccine it was shown that the IgM+IgD+CD27+ B cells that showed a major contribution to this TI stimulation were indeed pre–diversified and had acquired a high load of somatic IgV gene mutations in earlier immune responses, likely against gut–resident bacteria (94). These prior immune responses were likely TD immune responses involving GC reactions, as they also gave rise to numerous clonally related class–switched memory B cells (94). Indications for the reactivation of IgM+IgD+CD27+ memory B cells already present at the time of TI immunization was also provided in other studies (95, 96). Therefore, the frequent responsiveness of IgM+IgD+CD27+ B cells to pure polysaccharide antigens does not establish that these cells were generated in a TI response with SHM taking place outside of GC. It may well be that these cells were generated in earlier immune reactions in which polysaccharides as components of bacteria elicited TD immune responses.
Finally, a GC–independent SHM in the generation of mutated IgM+IgD+CD27+ B cells was also proposed based on the observation that patients with a deficiency in the inducible costimulator (ICOS) gene lacked class–switched memory B cells but showed normally mutated IgM+IgD+CD27+ B cells (97). However, small GC structures were detected in a lymph node available from one patient, and it is known from studies in the mouse that ICOS plays an important role in the induction of CSR (98). Thus, it may well be that the rudimentary GC in ICOS–deficient patients give rise to IgV gene mutated IgM+IgD+CD27+ memory B cells, but fail to generate class–switched memory B cells because of impaired class–switching.
Origin and characteristics of human IgM–expressing memory B cells
In the initial description of specific human peripheral blood IgM+ B–cell subsets with somatically mutated IgV genes and a proposed origin from GC reactions, two subsets were distinguished, namely IgM+IgD+CD27+ and IgM+IgDlow/–CD27+ (IgM–only) B cells (19, 99). The observation that HIGM1 patients with a germline deficiency of the CD40L gene have IgM+IgD+CD27+ but practically no IgM–only B cells was later taken as an argument that these are indeed distinct subsets with distinct origins (75). However, our own transcriptomic analysis of such cells revealed IgD as the only significantly differentially expressed gene (29), and cells of these subsets show a very similar BCR repertoire and often common B–cell clones (25). As IgM–only B cells have slightly higher IgV gene mutation frequencies than IgM+IgD+CD27+ B cells (25), it is likely that IgM–only B cells typically develop later in the GC response (i.e., after more rounds of proliferation and mutation) than the IgM+IgD+CD27+ B cells and that this is associated with a preferential downregulation of IgD. In disease situation such as the HIGM1 syndrome with an impaired GC reaction, the few memory B cells that are generated may not reach the differentiation stage when IgD is typically downregulated. As there is thus indication that the two types of IgM–expressing CD27+ memory B cells are largely identical, and as most studies did not distinguish between the large population of IgM+IgD+CD27+ and the rare IgM–only B cells, we focus the following discussion on IgM+IgD+CD27+ cells, but typically mean to include the IgM–only B cells.
Considering the ongoing debate about the origin and identity of IgM+(IgD+)CD27+ B cells, we discuss the characteristic features of these cells with a focus on the question whether these features argue for a derivation of these cells from TD immune responses and their memory B–cell identity.
First, the IgV mutation frequency of IgM+IgD+CD27+ B cells is very low in newborns and slowly accumulates with increasing age, as is also typical for classical class–switched memory B cells and as is expected for B cells that are generated in response to infections and vaccinations. Please note that the frequency of IgM+IgD+CD27+ B cells does typically not change with age, in contrast to class-switched memory B cells (Table 1) (8, 18, 24, 64, 100). One potential explanation for this is that very early in life GC reactions do not yet function optimally (e.g., because of an immaturity of TFH cells), and that CSR is less efficient, so that GC B cells preferentially differentiate into memory B cells without undergoing CSR.
Second, IgM+IgD+CD27+ B cells are long–lived cells, with a similar longevity as class–switched memory B cells (101), and persistence of IgM memory B–cell clones over years has been observed (102–104). This is a further hallmark of memory B cells. In some specific settings, the frequency of antigen–specific IgM+IgD+CD27+ B cells became reduced in peripheral blood faster than that of IgG+ memory B cells (105). However, this may be a specific feature of that particular immune response, and may involve a preferential homing of IgM+IgD+CD27+ B cells with time in secondary lymphoid organs, so that their frequency in peripheral blood is lowered, and/or a further differentiation of a fraction of IgM memory B cells into IgG memory B cells though CSR.
Third, transcriptomic analyses revealed that IgM+IgD+CD27+ B cells have a global gene expression pattern closely related to that of class–switched memory B cells, and clearly distinct from that of naive B cells (29, 31, 33). This similarity is also evident at the protein level, because IgM+IgD+CD27+ and class–switched B cells share a higher expression of several typical B–cell differentiation markers in comparison to naive B cells, including CD24, CD45RB (clone MEM–55), CD80, CD180, and TACI (38, 106), whereas the naive B–cell marker CD23 is neither expressed by IgM+IgD+CD27+ B cells nor by class–switched B cells (107). A further feature where IgM+IgD+CD27+ B cells show a higher global similarity to class–switched memory B cells than to naive B cells is the DNA methylation pattern (108). Hence, also at the epigenetic level, IgM+IgD+CD27+ B cells are similar to classical IgG+ memory B cells and substantially more different to pre–GC naive B cells.
Fourth, in vitro stimulation studies revealed that IgM+IgD+CD27+ B cells have the propensity to reacquire GC B–cell features, whereas IgG+ memory B cells preferentially differentiate into plasma cells upon restimulation (25, 29). This is reminiscent of the situation in mice, where transfer experiments revealed that GC–derived IgM+ memory B cells upon immunization establish new GC in recipient mice, whereas IgG+ memory B cells mainly differentiated into plasma cells (109, 110). These observations further support a link of human IgM+IgD+CD27+ B cells to the GC reaction.
Fifth, IgM+IgD+CD27+ and class–switched B cells are in vitro in numerous stimulation conditions easier to activate than naive B cells, and hence share this further hallmark of memory B cells with class–switched memory B cells (29–37).
Sixth, about 20% of IgM+IgD+CD27+ B cells and 30% of IgG memory B cells of human adults carry somatic mutations in the 5’ region of the BCL6 gene (60). BCL6 is an off–target for the SHM process and acquires mutations in about 30% of GC B cells, at a frequency about one fifties of the IGHV gene mutation frequency of the same cells (111). As somatic hypermutation is strictly dependent on active transcription of its target genes (112–114), and as BCL6 is only transcribed at substantial levels in GC B cells (115, 116), this argues for a GC derivation of IgM+IgD+CD27+ B cells. The lower frequency of BCL6–mutated IgM+IgD+CD27+ than IgG+ B cells is to be expected, because the non–class–switched cells also have a similarly lower IGHV gene mutation frequency, likely due to their typically earlier exit from the GC (19). Although this genetic feature of IgM+IgD+CD27+ B cells cannot clarify whether all of these cells are GC–experienced, the frequency of BCL6–mutated IgM+IgD+CD27+ B cells nevertheless argues that at least a large fraction of these cells derives from GC B cells.
Seventh, IgM+IgD+CD27+ and class–switched memory B cells show further similarities for two features of their BCR structure and repertoire: The IGHV region genes of class–switched and IgM+IgD+CD27+ B cells have on average shorter CDRIII lengths than naive B cells (23, 38, 65, 117–119), and both B–cell subsets show a counterselection of usage of the IGHV4–34 gene (23, 38, 65, 119). As IGHV4–34 is inherently autoreactive (120), and as also BCRs with long CDRIII tend to be poly–/autoreactive (121), it is thought that the counterselection against such potentially dangerous features occurs during the GC reaction and is hence a feature of post–GC memory B cells (38, 120, 121). However, in several studies, the overall BCR repertoire in terms of IGHV gene usage of IgM+IgD+CD27+, IgM–only and class–switched memory B cells showed relatively little overlap, and this was taken as an indication that these subsets have a distinct origin (27, 28, 117). A possible explanation for this can be that in many immune responses, preferentially either class–switched or IgM memory B cells are generated, so that the overall repertoires appear distinct, even though both subsets derive from GC reactions. Similarly, the larger number of distinct clones among IgM+IgD+CD27+ B cells compared to class–switched memory B cells in young children (85) may reflect that there is less efficient CSR in GC in the first two years of life, so that a polyclonal pool of non–class–switched memory B cells is already established when IgG+ memory B cells are still few and derive from fewer clones.
Eighth, a further, and perhaps the key genetic characteristic feature of GC–derived memory B cells is the presence of somatic mutations in the rearranged IgV genes of nearly all of these cells. This is also true for IgM+IgD+CD27+ B cells, which show an average IGHV mutation frequency of 4–5% in adults (19, 23, 25). As the GC is the only known structure where substantial levels of SHM are acquired in humans (Box 1), this strongly argues for a GC derivation of most if not all IgM+IgD+CD27+ B cells, at least in adults.
Nineth, and related to the prior feature, we and others identified numerous large memory B–cell clones which were composed of IgM+IgD+CD27+ and class–switched B cells (25, 60, 94, 100, 103, 104, 117, 122). The hierarchical trees generated from the mutated IgV gene sequences frequently showed an intermingling of IgM– and IgG–expressing B cells, which is a strong argument that these clone members derive from a common mutating GC B–cell clone. Notably, IgM+IgD+CD27+ B cells often were closer to the root of the trees than class–switched memory B cells (25, 60). This fits very well to the observation that the IgV genes of IgM+IgD+CD27+ B cells are on average less mutated than class–switched memory B cells and explains this by indicating that the IgM–expressing memory B cells typically exit the GC after fewer rounds of proliferation and mutation than IgG+ and IgA+ B cells, and have hence acquired less mutations. Clonal relationships between IgM– and IgG–expressing B cells were in some studies only rarely or not at all observed (27, 28). However, one has to consider that in most studies only a relatively small number of B cells was studied, considering the size of the overall B–cell pool of an individual. Also in our own studies, there was a clear tendency that the chances to identify common clone members among IgM+ and class–switched memory B cells was higher when the sample size or the overall size of a clone was larger (25). In further support of a GC derivation of IgM+IgD+CD27+ B cells, in a study that included deep sequencing of IgV genes from intestinal GC and from memory B–cells, common clones of intestinal GC B cells and IgM+IgD+CD27+ B cells with intermingling mutation patterns were identified (123).
Box 1. Considerations for SHM outside GC or in TI GC responses
Considering the hypothesis of SHM in human B cells taking place outside of the GC, there are indeed AID–expressing B cells detectable outside of GC in secondary lymphoid organs (89, 90). However, these are singular cells, and it is likely that these are B cells induced to undergo CSR, which is also dependent on AID expression. It cannot be excluded that some AID–expressing B cells outside GC acquire IgV gene point mutations. However, one has to consider that IgM+IgD+CD27+ B cells in adolescents and adults carry a substantial level of somatic mutations in the IGH and IGL V genes (4% on average), and that most mutations are disadvantageous. This therefore argues that a stepwise process of proliferation, somatic hypermutation and selection as in the GC is needed to generate memory B cells with often more than ten point mutations per IgV gene and a functional and antigen–specific BCR. Importantly, clusters of AID–expressing B cells were so far not detected outside of GC in humans (89–91), so that it remains enigmatic where and how well–mutated IgM+IgD+CD27+ B cells would obtain their IgV gene mutations outside GC.
A further source of IgM+ memory B cells could potentially be TI immune responses that involve GC–like structures. There are indeed reports that immunizations of mice with TI antigens can induce GC–like structure, and that some of these GC B cells can develop into memory B cells (92). However, these GC–like structures developing in the absence of T cells are short–lived and vanish after a few days (92, 93). Therefore, it is highly unlikely that such structures can give rise to well mutated memory B cells, such as human IgM+IgD+CD27+ B cells. Moreover, it seems that GC without any TFH cells have not been identified in healthy humans.
Tenth, in a number of specific infections and immunizations, antigen–specific IgM+IgD+CD27+ memory B cells were identified besides class–switched memory B cells, and sometimes they were even the dominant memory B–cell subset. This includes immune responses against rotavirus (124, 125), hepatitis B virus (126), papilloma virus (127), polyomavirus (128), Rhesus D blood group antigen (129), SARS–CoV–2 (102), tetanus toxoid (129, 130), Streptococcus pneumoniae (95), and Plasmodium falciparum (131). This clearly shows that in these typical TD immune responses, the memory B–cell compartment also encompasses IgM+ memory B cells.
While the multiple characteristic features of IgM+IgD+CD27+ B cells discussed above in our view strongly argues for their GC derivation and memory B–cell identity, it remains unclear how to reconcile this with the indications for a GC–independent generation of these cells. Considering that most studies supporting the idea of a GC– and/or T cell–independent origin were focused on such B cells in children, whereas most studies supporting a GC–derivation of IgM+IgD+CD27+ B cells were performed with B cells from human adults, one may speculate that there might be different developmental pathways for these B cells active in early childhood versus adulthood, and that IgM+IgD+CD27+ B cells are not a homogenous population but may indeed encompass distinct subsets. A direct analysis of this idea was recently published by Grimsholm and colleagues (65). They distinguished two subsets of IgM+IgD+CD27+ B cells based on the expression level of CD27 (65). In very young children a CD27dullIgM+IgD+ B–cell population with lowly mutated IgV genes dominated, whereas with increasing age, CD27brightIgM+IgD+ B cells with higher mutation loads predominated (61, 65). In patients with immunodeficiencies that are expected to impair GC responses (e.g., HIGM1), CD27brightIgM+IgD+ B cells were missing, but CD27dullIgM+IgD+ B cells were present, leading the authors to conclude that the CD27dullIgM+IgD+ B cells can be generated in a GC independent way, whereas CD27brightIgM+IgD+ B cells are post–GC memory B cells (65).
Taken together, there is now strong evidence that the vast majority of human peripheral blood IgM+IgD+CD27+ B cells in adults are post–GC memory B cells, but in younger children a considerable subset of these cells may derived from a so far poorly understood distinct developmental pathway, which may not involve conventional GC reactions.
Splenic marginal zone B cells
The human spleen is the largest secondary organ of the immune system and has important functions in blood filtration, iron metabolism, and immune defense, and is a major reservoir of lymphocytes (132). The splenic marginal zone (sMZ) is a unique area of reduced blood–flow that allows close contact of blood–borne antigens or pathogens with specialized immune cells (133). Thereby, the cells of the sMZ provide immunosurveillance of the peripheral blood.
In order to fulfill their important function in the first line of defense against pathogens invading the bloodstream, sMZ B cells require additional signals from bystander cells in their microenvironment (134, 135). Multiple studies have provided insight into the crosstalk among human sMZ B cells and innate immune cells, such as dendritic cells, natural killer cells, neutrophils, and several types of stromal cells (136–142). These cells provide strong signals via BAFF, APRIL, and DLL1 (141, 143, 144). Human sMZ B cells seem to be less dependent on canonical T–cell help (e.g., through CD40L) and are highly dependent on microbial signals for their recruitment or homeostasis (75, 85, 145). In addition, the literature supports the view that gut microbiota–derived antigens might play a role in sMZ B–cell homeostasis in humans (123, 146–149). These observations support a role of sMZ B cells in TI immune responses, but with substantial help from various innate immune cells. It has been reported that interaction of sMZ B cells with neutrophils in vitro induces SHM in their IgV genes (137). However, the increased somatic mutation load seen in the BCRs may simply represent preferential survival and/or outgrowth of (more highly) mutated B cells in the prolonged cultures.
The complex crosstalk in the human sMZ is thought to recruit circulating B cells and to induce a primed state (135). The reprogramming of circulating B cells into the sMZ B–cell compartment does not favor one specific B–cell subset, but naive, IgM memory as well as class–switched memory B cells are susceptible to DLL1–NOTCH2–dependent in vitro induction of the sMZ B–cell phenotype and gene expression profile (100, 150). However, in adults, the vast majority of sMZ B cells harbor mutated IgV genes and already in young children the sMZ precursor population expresses CD45RBMEM55, which is a memory B cell marker (150, 151). This indicates that memory B cells have an intrinsic advantage over naive B cells in homing to the sMZ and undergoing reprogramming to sMZ B cells (Figure 3).
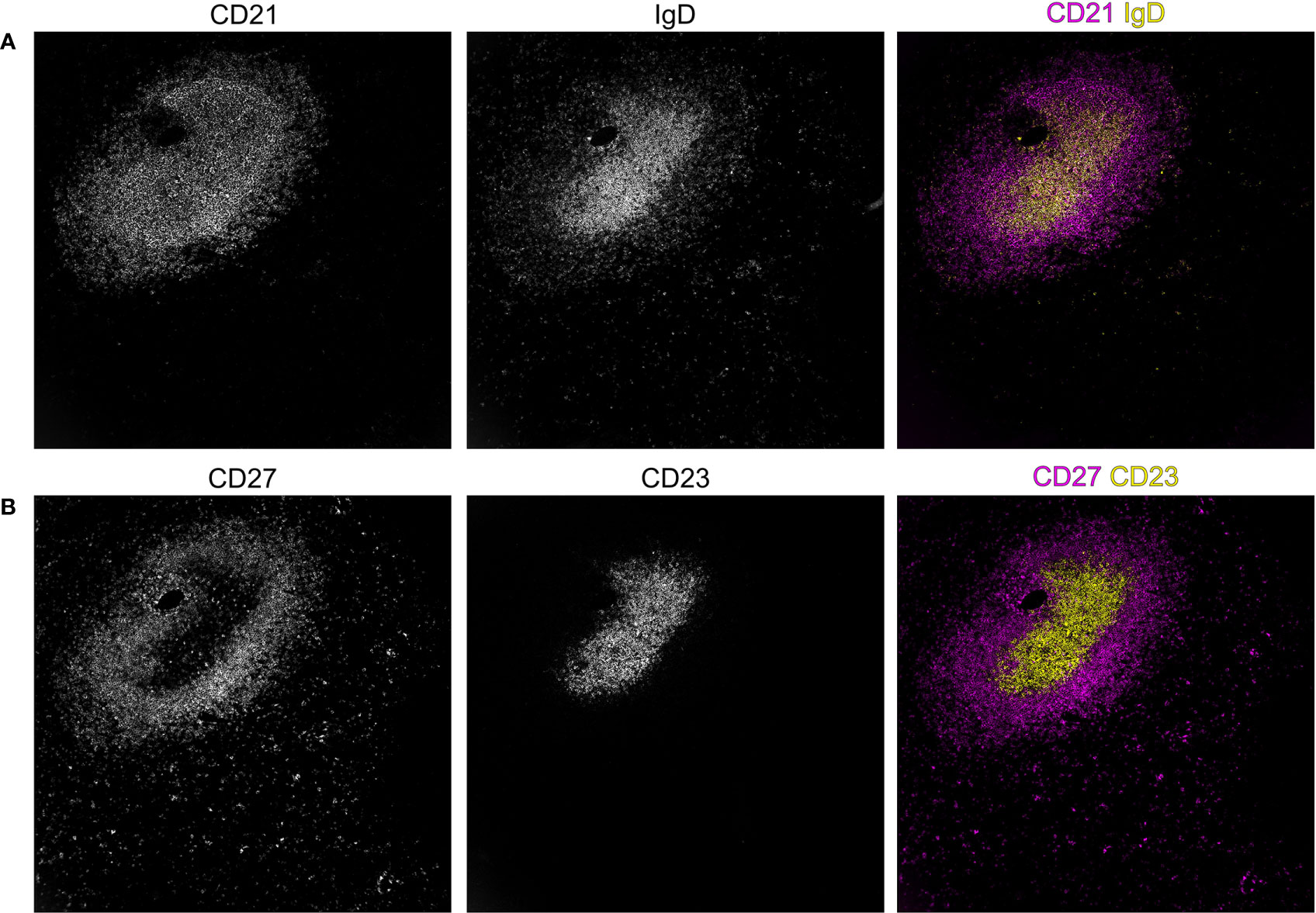
Figure 3 Spatial distribution of B-cell subsets in the splenic white pulp of an adult human individual. In secondary lymphoid organs, such as the spleen, B–cell subsets segregate to different compartments as can be shown by the expression of the markers IgD, CD21, CD23, and CD27. The staining of CD21 shows the B–cell follicle, revealing a compartment of CD21high follicular dendritic cells (FDC), a population of fibroblasts that supports adaptive immunity in the germinal center reaction by its unique capability to acquire and present antigen to B cells in its native form. In addition to the dense area of CD21high FDCs, a more superficial population of CD21 expressing cells is visible in (A), highlighting the B cells of the sMZ, which also co-express the memory B–cell marker CD27 (B) in adults. In contrast, (A) IgD– and (B) CD23–expressing cells are confined to the center of the follicular region. Stainings were performed with the MACSima platform (Miltenyi Biotech, Bergisch Gladbach, German). The dimensions of the images are 750 x 750 µm.
Human sMZ B cells were defined as the human counterpart of murine sMZ B cells by their homologous localization in the microanatomical niche at the interface of splenic B–cell follicles and the red pulp (152). Murine sMZ B cells and human sMZ B cells furthermore share functional propensities and express similar phenotypes, e.g., high expression of CD21 and IgM, but low expression of IgD (136, 138, 153). Furthermore, signaling through the NOTCH2 receptor seems to be essential for the generation of sMZ B cells not only in the mouse but likely also in humans (100, 154). However, there are also significant interspecies differences between humans and rodents in regard to the organization of the white pulp, the structure of the sMZ (133, 155), the phenotype and IgV gene mutation status of sMZ B cells in adult individuals (135, 153, 156), as well as their functional capacities, which caused dispute about the identity and memory character of human sMZ B cells (85, 157). A more detailed comparison of human and murine sMZ B cells was already covered in multiple publications (100, 134, 135, 155, 158).
Importantly, rodent sMZ B cells mostly carry unmutated IgV genes (159), although there is indication that these cells also encompass a subset of memory B cells from TD immune responses (160). In distinction to this, in humans, as discussed in the previous chapter in the case of blood-derived IgM+IgD+CD27+ B cells, responsiveness to TD antigens, CD27-expression and IgV-gene and BCL6 mutations indicate a GC-derivation of human sMZ B cells (64, 85, 100, 153, 156, 161). BCR repertoire deep sequencing analyses point to the existence of a body–wide clonal network, which interconnects sMZ B cells with memory B–cell clone members in distant lymphoid organs and supports the view that human sMZ B cells can undergo subsequent GC reactions and recirculate to distant organs via the blood stream (88, 162–164). It was also observed that many circulating memory B cells are clonally related to sMZ B cells, supporting the idea of a systematic memory B–cell archive in the spleen (134).
Besides the indications for a derivation of at least a large fraction of sMZ B cells from TD immune responses, there are also indications for a generation of sMZ B cells in TI immune responses. Pioneering studies have established that the susceptibility to infections with encapsulated bacteria upon splenectomy is a consequence of the concomitant loss of sMZ B cells (84, 87) and their specialized microanatomical niche, which is strategically placed at the border of the splenic immune compartment and the circulatory compartment. SMZ B cells show unique response kinetics upon stimulation and their frontline localization allows sMZ B cells and sMZ–resident phagocytes to bind and remove circulating pathogens from the bloodstream through the induction of TI antibody responses (38, 75, 135, 137, 165).
Intriguingly, not only splenectomy renders individuals more susceptible to bacterial infections, which are thought to often cause TI immune responses, but also age is a critical determinant of splenic immune function: Newborn, young children, and seniors are at increased risk of suffering from severe infections with encapsulated bacteria (166, 167). It was suggested that the vulnerability in young individuals is a result of incomplete maturation of the sMZ B–cell compartment (168, 169). Our own results showed that sMZ B cells are already detectable only two weeks after birth and show equivalent response dynamics as adult–derived sMZ B cells, however, lacking IgV gene mutations and a compartment of class–switched sMZ B cells (64, 100). This indicates that a large fraction of these sMZ B cells in very young individuals have only limited or no GC experience.
There were no significant differences regarding CDRIII length distribution, IgV gene usage, and IgV gene mutation load when comparing the BCR repertoire of paired sMZ B cells to non–sMZ B cells derived from spleen and blood of the same individual. This finding indicates that the sampling process of splenic B cells into the sMZ compartment is largely random (64). In addition, the spleen seems to induce a distinct phenotype among memory B cells, which differs from the equivalent B–cell subsets in blood and other secondary lymphoid organs, e.g., in higher expression of CD21, CCR7, and CD180 by sMZ B cells, or a higher fraction of CD69– and CD45RB–expressing cells (123, 170). These phenotypical adaptations of splenic B cells might contribute to immune protection against blood–borne pathogens.
In seniors, splenic naive B cells are virtually absent and the fraction of class–switched sMZ B cells that harbor highly mutated IgV genes is massively increased. Moreover, few, extremely large, mostly class–switched B–cell clones dominate the splenic BCR repertoire at the expense of smaller clones in the elderly (100). This skewed composition of the splenic B–cell pool in elderly individuals possibly contributes to the diminished efficiency of immune responses against newly encountered pathogens, vaccinations, and polysaccharide antigens in old age (166, 167).
Taken together, the sMZ B–cell compartment seems to change substantially during the life of humans and there is ample evidence that these B cells may either derive from TI or TD immune responses, and may also have functions in both types of immune responses. This is indeed important to distinguish, because it is well known that GC–derived memory B cells may be reactivated without T cell help (94, 96), so an engagement of sMZ in TI immune responses as such does not argue that the cells were not originally generated in GC reactions of TD responses. A possible solution to the seemingly contradictory origins of sMZ B cells could be that the cells are not a homogenous population, but that they include distinct B–cell subsets with distinct origins. There are indeed indications for a heterogeneity of these cells (64, 100, 123). A mass cytometry and single cell RNA–sequencing study identified two subsets of human sMZ B cells, one with unmutated or lowly mutated IgV genes and further characteristics of a GC–independent generation (termed MZB–2), and the other with well mutated IgV genes and further memory B–cell features (e.g., higher CD27 expression; MZB–1) (147). The CD27low MZB–2 subset was a minor subset in adults. Notably, the MZB–1 cells were frequently clonally related to IgM–only B cells (147), a bona–fide post–GC memory B–cell population, as discussed above. The identification of a specific human sMZ precursor population that is frequent early in life but diminishes with increasing age (150), fits to the concept that in young children the sMZ is mainly populated by GC–inexperienced (but nevertheless perhaps often memory) B cells that are also mainly involved in TI immune responses. With increasing age these cells are continuously replaced by post–GC, in young children mostly IgM–expressing, but in older children and adolescents also IgG+ memory B cells that can be engaged in both TI and TD immune responses.
Concluding remarks
Human IgM+IgD+CD27+ and IgM–only B cells together account for about 20% of the B-cell pool in human adults, so that it is clearly highly relevant to know the origin and function of these cells for a comprehensive understanding of humoral immunity in humans. After more than 20 years of controversial discussions about the origin and function of these IgM–expressing B cells with somatically mutated IgV genes (19, 75, 99), now a scenario is developing which may resolve the controversies and provide an integrative view on the numerous studies on this topic. Based on studies either focused on B cells in the peripheral blood or those in the spleen, it seems that early in life, some IgM+IgD+ B cells may undergo a low level of SHM outside of GC reactions and TD immune responses. While independent studies of fetal or early life B cells or B cells from patients with particular immunodeficiencies and single cell transcriptomic studies of splenic B cells support this concept (65, 75, 76, 78, 147, 150), it is still unresolved where in the human body SHM takes place outside of GC, and to what extent these early life lowly IgV mutated IgM+IgD+CD27+ B cells derive from either an antigen–independent primary diversification process or from TI immune responses. Soon after birth, when children are exposed to a myriad of foreign antigens driving TD immune responses, a population of IgM+IgD+CD27+ B cells emerges with all hallmark features of post–GC TD memory B cells: longevity, well mutated IgV genes, pathogen specificity, fast and easy response kinetics, and expanded B–cell clones. In the adult, these post–GC IgM+IgD+CD27+ memory B cells represent the vast majority of the IgM+IgD+CD27+ B cells in peripheral blood and the sMZ (19, 65, 75, 99, 147), so the contributions of the early life non–GC derived IgM+IgD+CD27+ B cells become negligible. Direct evidence for two distinct subsets of IgM+IgD+CD27+ B cells both in peripheral blood and in the spleen, with one showing indications for a GC independent generation and dominating early in life, and the other with key post–GC memory B–cell features dominating in adulthood, strongly support this scenario (64, 65, 147).
Regarding the numerous features brought forward earlier in the review to argue for a GC derivation of IgM+IgD+CD27+ B cells in adults, we consider some arguments as particularly strong. This includes the detection of shared clones with intermingling IgV gene mutation patterns with classical class–switched memory B cells (25, 60, 94, 100, 103, 117, 122), the presence of BCL6 mutations in a considerable fraction of these cells (60, 161), and the existence of long–lived IgM+IgD+CD27+ B cells among pathogen–specific memory B cells for numerous viral and bacterial species that elicit TD humoral immune responses (95, 102, 124–131). Other features of these cells in common with class–switched memory B cells, such as the transcriptomes, specific BCR repertoire features, surface marker expression patterns, and in vitro reactivities, may be primarily regarded as only correlative and might also be features of the proposed memory B cells derived from TI responses. However, in our view, the multitude of common characteristic of IgM+IgD+CD27+ B cells with GC–derived IgG+ and IgA+ memory B cells indeed strongly supports a common origin of these cells from GC reactions. The advantage for the immune system to have distinct subsets of memory B cells is obvious: The more highly IgV mutated class–switched memory B cells can quickly differentiate into plasma cells when encountering again the specific pathogens that led to their generation for a fast increase in specific antibody production. IgM+IgD+CD27+ B cells can also undergo such differentiation and can become IgM–secreting plasma cells, which conveys highly efficient complement fixation. Importantly, IgM+IgD+CD27+ B cells additionally have the propensity upon re–encounter of the same or a related antigen to again enter GC reactions, to adopt the BCR specificity to a potentially altered antigen through new rounds of SHM and selection (2). These IgM–expressing memory B cells also have the flexibility to switch to a particular IgG or IgA class, depending on what is needed for the secondary immune response. They are thus a particularly flexible arm of our immunological memory.
Author contributions
BB: Conceptualization, Writing – original draft, Writing – review & editing. AK: Conceptualization, Writing – original draft, Writing – review & editing. RK: Conceptualization, Writing – original draft, Writing – review & editing.
Funding
The author(s) declare financial support was received for the research, authorship, and/or publication of this article. Own work discussed in this review was supported by the Deutsche Forschungsgemeinschaft (KU1315/10–1, KU1315/14–1, KU1315/16–1), the Wilhelm Sander Stiftung (2018.101.1), the German José Carreras Leukemia Foundation (DJCSL02 R/2020), and the BIOME postdoctoral excellence program of the Medical Faculty of the University of Duisburg–Essen. We acknowledge support by the Open Access Publication Fund of the University of Duisburg-Essen.
Acknowledgments
We thank Marc Seifert and Marc Weniger for many stimulating discussions. We thank Aparajita Singh for her support with MACSima high content imaging of human spleens.
Conflict of interest
The authors declare that the research was conducted in the absence of any commercial or financial relationships that could be construed as a potential conflict of interest.
Publisher’s note
All claims expressed in this article are solely those of the authors and do not necessarily represent those of their affiliated organizations, or those of the publisher, the editors and the reviewers. Any product that may be evaluated in this article, or claim that may be made by its manufacturer, is not guaranteed or endorsed by the publisher.
References
1. Victora GD, Nussenzweig MC. Germinal centers. Annu Rev Immunol (2012) 30:429–57. doi: 10.1146/annurev-immunol-020711-075032
2. Seifert M, Küppers R. Human memory B cells. Leukemia (2016) 30:2283–92. doi: 10.1038/leu.2016.226
3. Korzhenevich J, Janowska I, van der Burg M, Rizzi M. Human and mouse early B cell development: So similar but so different. Immunol Lett (2023) 261:1–12. doi: 10.1016/j.imlet.2023.07.004
4. Agrawal A, Schatz DG. RAG1 and RAG2 form a stable postcleavage synaptic complex with DNA containing signal ends in V(D)J recombination. Cell (1997) 89:43–53. doi: 10.1016/s0092-8674(00)80181-6
5. Matsuda F, Honjo T. Organization of the human immunoglobulin heavy-chain locus. Adv Immunol (1996) 62:1–29. doi: 10.1016/s0065-2776(08)60426-5
6. Imkeller K, Wardemann H. Assessing human B cell repertoire diversity and convergence. Immunol Rev (2018) 284:51–66. doi: 10.1111/imr.12670
7. Rajewsky K. Clonal selection and learning in the antibody system. Nature (1996) 381:751–8. doi: 10.1038/381751a0
8. Budeus B, Kibler A, Brauser M, Homp E, Bronischewski K, Ross JA, et al. Human cord blood B cells differ from the adult counterpart by conserved Ig repertoires and accelerated response dynamics. J Immunol (2021) 206:2839–51. doi: 10.4049/jimmunol.2100113
9. Garside P, Ingulli E, Merica RR, Johnson JG, Noelle RJ, Jenkins MK. Visualization of specific B and T lymphocyte interactions in the lymph node. Science (1998) 281:96–9. doi: 10.1126/science.281.5373.96
10. Taylor JJ, Pape KA, Jenkins MK. A germinal center-independent pathway generates unswitched memory B cells early in the primary response. J Exp Med (2012) 209:597–606. doi: 10.1084/jem.20111696
11. Küppers R, Zhao M, Hansmann ML, Rajewsky K. Tracing B cell development in human germinal centres by molecular analysis of single cells picked from histological sections. EMBO J (1993) 12:4955–67. doi: 10.1002/j.1460-2075.1993.tb06189.x
12. Liu YJ, Zhang J, Lane PJ, Chan EY, MacLennan IC. Sites of specific B cell activation in primary and secondary responses to T cell-dependent and T cell-independent antigens. Eur J Immunol (1991) 21:2951–62. doi: 10.1002/eji.1830211209
13. Muramatsu M, Kinoshita K, Fagarasan S, Yamada S, Shinkai Y, Honjo T. Class switch recombination and hypermutation require activation-induced cytidine deaminase (AID), a potential RNA editing enzyme. Cell (2000) 102:553–63. doi: 10.1016/s0092-8674(00)00078-7
14. Victora GD, Schwickert TA, Fooksman DR, Kamphorst AO, Meyer-Hermann M, Dustin ML, et al. Germinal center dynamics revealed by multiphoton microscopy with a photoactivatable fluorescent reporter. Cell (2010) 143:592–605. doi: 10.1016/j.cell.2010.10.032
15. Weisel FJ, Zuccarino-Catania GV, Chikina M, Shlomchik MJ. A temporal switch in the germinal center determines differential output of memory B and plasma cells. Immunity (2016) 44:116–30. doi: 10.1016/j.immuni.2015.12.004
16. King HW, Orban N, Riches JC, Clear AJ, Warnes G, Teichmann SA, et al. Single-cell analysis of human B cell maturation predicts how antibody class switching shapes selection dynamics. Sci Immunol (2021) 6:eabe6291. doi: 10.1126/sciimmunol.abe6291
17. Roco JA, Mesin L, Binder SC, Nefzger C, Gonzalez-Figueroa P, Canete PF, et al. Class-switch recombination occurs infrequently in germinal centers. Immunity (2019) 51:337–50 e7. doi: 10.1016/j.immuni.2019.07.001
18. Agematsu K, Nagumo H, Yang FC, Nakazawa T, Fukushima K, Ito S, et al. B cell subpopulations separated by CD27 and crucial collaboration of CD27+ B cells and helper T cells in immunoglobulin production. Eur J Immunol (1997) 27:2073–9. doi: 10.1002/eji.1830270835
19. Klein U, Rajewsky K, Küppers R. Human immunoglobulin (Ig)M+IgD+ peripheral blood B cells expressing the CD27 cell surface antigen carry somatically mutated variable region genes: CD27 as a general marker for somatically mutated (memory) B cells. J Exp Med (1998) 188:1679–89. doi: 10.1084/jem.188.9.1679
20. Jung J, Choe J, Li L, Choi YS. Regulation of CD27 expression in the course of germinal center B cell differentiation: the pivotal role of IL-10. Eur J Immunol (2000) 30:2437–43. doi: 10.1002/1521-4141(2000)30:8<2437::AID-IMMU2437>3.0.CO;2-M
21. Odendahl M, Jacobi A, Hansen A, Feist E, Hiepe F, Burmester GR, et al. Disturbed peripheral B lymphocyte homeostasis in systemic lupus erythematosus. J Immunol (2000) 165:5970–9. doi: 10.4049/jimmunol.165.10.5970
22. Fecteau JF, Cote G, Neron S. A new memory CD27-IgG+ B cell population in peripheral blood expressing VH genes with low frequency of somatic mutation. J Immunol (2006) 177:3728–36. doi: 10.4049/jimmunol.177.6.3728
23. Tucci FA, Kitanovski S, Johansson P, Klein-Hitpass L, Kahraman A, Durig J, et al. Biased IGH VDJ gene repertoire and clonal expansions in B cells of chronically hepatitis C virus-infected individuals. Blood (2018) 131:546–57. doi: 10.1182/blood-2017-09-805762
24. Blanco E, Perez-Andres M, Arriba-Mendez S, Contreras-Sanfeliciano T, Criado I, Pelak O, et al. Age-associated distribution of normal B-cell and plasma cell subsets in peripheral blood. J Allergy Clin Immunol (2018) 141:2208–19 e16. doi: 10.1016/j.jaci.2018.02.017
25. Budeus B, Schweigle de Reynoso S, Przekopowitz M, Hoffmann D, Seifert M, Küppers R. Complexity of the human memory B-cell compartment is determined by the versatility of clonal diversification in germinal centers. Proc Natl Acad Sci USA (2015) 112:E5281–9. doi: 10.1073/pnas.1511270112
26. Wu YC, Kipling D, Dunn-Walters DK. Age-related changes in human peripheral blood IGH repertoire following vaccination. Front Immunol (2012) 3:193. doi: 10.3389/fimmu.2012.00193
27. Wu YC, Kipling D, Leong HS, Martin V, Ademokun AA, Dunn-Walters DK. High-throughput immunoglobulin repertoire analysis distinguishes between human IgM memory and switched memory B-cell populations. Blood (2010) 116:1070–8. doi: 10.1182/blood-2010-03-275859
28. Wu YC, Kipling D, Dunn-Walters DK. The relationship between CD27 negative and positive B cell populations in human peripheral blood. Front Immunol (2011) 2:81. doi: 10.3389/fimmu.2011.00081
29. Seifert M, Przekopowitz M, Taudien S, Lollies A, Ronge V, Drees B, et al. Functional capacities of human IgM memory B cells in early inflammatory responses and secondary germinal center reactions. Proc Natl Acad Sci USA (2015) 112:E546–55. doi: 10.1073/pnas.1416276112
30. Shi Y, Agematsu K, Ochs HD, Sugane K. Functional analysis of human memory B-cell subpopulations: IgD+CD27+ B cells are crucial in secondary immune response by producing high affinity IgM. Clin Immunol (2003) 108:128–37. doi: 10.1016/s1521-6616(03)00092-5
31. Good KL, Avery DT, Tangye SG. Resting human memory B cells are intrinsically programmed for enhanced survival and responsiveness to diverse stimuli compared to naive B cells. J Immunol (2009) 182:890–901. doi: 10.4049/jimmunol.182.2.890
32. Good KL, Bryant VL, Tangye SG. Kinetics of human B cell behavior and amplification of proliferative responses following stimulation with IL-21. J Immunol (2006) 177:5236–47. doi: 10.4049/jimmunol.177.8.5236
33. Good KL, Tangye SG. Decreased expression of Kruppel-like factors in memory B cells induces the rapid response typical of secondary antibody responses. Proc Natl Acad Sci USA (2007) 104:13420–5. doi: 10.1073/pnas.0703872104
34. Bernasconi NL, Traggiai E, Lanzavecchia A. Maintenance of serological memory by polyclonal activation of human memory B cells. Science (2002) 298:2199–202. doi: 10.1126/science.1076071
35. Maurer D, Fischer GF, Fae I, Majdic O, Stuhlmeier K, Von Jeney N, et al. IgM and IgG but not cytokine secretion is restricted to the CD27+ B lymphocyte subset. J Immunol (1992) 148:3700–5. doi: 10.4049/jimmunol.148.12.3700
36. Tangye SG, Avery DT, Deenick EK, Hodgkin PD. Intrinsic differences in the proliferation of naive and memory human B cells as a mechanism for enhanced secondary immune responses. J Immunol (2003) 170:686–94. doi: 10.4049/jimmunol.170.2.686
37. Tangye SG, Avery DT, Hodgkin PD. A division-linked mechanism for the rapid generation of Ig-secreting cells from human memory B cells. J Immunol (2003) 170:261–9. doi: 10.4049/jimmunol.170.1.261
38. Berkowska MA, Driessen GJ, Bikos V, Grosserichter-Wagener C, Stamatopoulos K, Cerutti A, et al. Human memory B cells originate from three distinct germinal center-dependent and -independent maturation pathways. Blood (2011) 118:2150–8. doi: 10.1182/blood-2011-04-345579
39. Mörbe UM, Jorgensen PB, Fenton TM, von Burg N, Riis LB, Spencer J, et al. Human gut-associated lymphoid tissues (GALT); diversity, structure, and function. Mucosal Immunol (2021) 14:793–802. doi: 10.1038/s41385-021-00389-4
40. Macpherson AJ, McCoy KD, Johansen FE, Brandtzaeg P. The immune geography of IgA induction and function. Mucosal Immunol (2008) 1:11–22. doi: 10.1038/mi.2007.6
41. Bergqvist P, Stensson A, Lycke NY, Bemark M. T cell-independent IgA class switch recombination is restricted to the GALT and occurs prior to manifest germinal center formation. J Immunol (2010) 184:3545–53. doi: 10.4049/jimmunol.0901895
42. Reboldi A, Arnon TI, Rodda LB, Atakilit A, Sheppard D, Cyster JG. IgA production requires B cell interaction with subepithelial dendritic cells in Peyer's patches. Science (2016) 352:aaf4822. doi: 10.1126/science.aaf4822
43. Lindner C, Thomsen I, Wahl B, Ugur M, Sethi MK, Friedrichsen M, et al. Diversification of memory B cells drives the continuous adaptation of secretory antibodies to gut microbiota. Nat Immunol (2015) 16:880–8. doi: 10.1038/ni.3213
44. Lindner C, Wahl B, Fohse L, Suerbaum S, Macpherson AJ, Prinz I, et al. Age, microbiota, and T cells shape diverse individual IgA repertoires in the intestine. J Exp Med (2012) 209:365–77. doi: 10.1084/jem.20111980
45. Seifert M, Steimle-Grauer SA, Goossens T, Hansmann ML, Bräuninger A, Küppers R. A model for the development of human IgD-only B cells: Genotypic analyses suggest their generation in superantigen driven immune responses. Mol Immunol (2009) 46:630–9. doi: 10.1016/j.molimm.2008.07.032
46. Reed DJ, Hawley J, Dang T, Yuan D. Role of differential mRNA stability in the regulated expression of IgM and IgD. J Immunol (1994) 152:5330–6. doi: 10.4049/jimmunol.152.11.5330
47. Liu YJ, de Bouteiller O, Arpin C, Briere F, Galibert L, Ho S, et al. Normal human IgD+IgM- germinal center B cells can express up to 80 mutations in the variable region of their IgD transcripts. Immunity (1996) 4:603–13. doi: 10.1016/s1074-7613(00)80486-0
48. Rowe DS, Fahey JL. A new class of human immunoglobulins. I. @ a unique myeloma protein. J Exp Med (1965) 121:171–84. doi: 10.1084/jem.121.1.171
49. Kluin PM, Kayano H, Zani VJ, Kluin-Nelemans HC, Tucker PW, Satterwhite E, et al. IgD class switching: identification of a novel recombination site in neoplastic and normal B cells. Eur J Immunol (1995) 25:3504–8. doi: 10.1002/eji.1830251244
50. Arpin C, de Bouteiller O, Razanajaona D, Fugier-Vivier I, Briere F, Banchereau J, et al. The normal counterpart of IgD myeloma cells in germinal center displays extensively mutated IgVH gene, Cmu-Cdelta switch, and lambda light chain expression. J Exp Med (1998) 187:1169–78. doi: 10.1084/jem.187.8.1169
51. Goossens T, Bräuninger A, Klein U, Küppers R, Rajewsky K. Receptor revision plays no major role in shaping the receptor repertoire of human memory B cells after the onset of somatic hypermutation. Eur J Immunol (2001) 31:3638–48. doi: 10.1002/1521-4141(200112)31:12<3638::aid-immu3638>3.0.co;2-g
52. Berkowska MA, Heeringa JJ, Hajdarbegovic E, van der Burg M, Thio HB, van Hagen PM, et al. Human IgE(+) B cells are derived from T cell-dependent and T cell-independent pathways. J Allergy Clin Immunol (2014) 134:688–97 e6. doi: 10.1016/j.jaci.2014.03.036
53. Gould HJ, Sutton BJ. IgE in allergy and asthma today. Nat Rev Immunol (2008) 8:205–17. doi: 10.1038/nri2273
54. He JS, Meyer-Hermann M, Xiangying D, Zuan LY, Jones LA, Ramakrishna L, et al. The distinctive germinal center phase of IgE+ B lymphocytes limits their contribution to the classical memory response. J Exp Med (2013) 210:2755–71. doi: 10.1084/jem.20131539
55. He JS, Subramaniam S, Narang V, Srinivasan K, Saunders SP, Carbajo D, et al. IgG1 memory B cells keep the memory of IgE responses. Nat Commun (2017) 8:641. doi: 10.1038/s41467-017-00723-0
56. Yang Z, Sullivan BM, Allen CD. Fluorescent in vivo detection reveals that IgE(+) B cells are restrained by an intrinsic cell fate predisposition. Immunity (2012) 36:857–72. doi: 10.1016/j.immuni.2012.02.009
57. Haniuda K, Fukao S, Kodama T, Hasegawa H, Kitamura D. Autonomous membrane IgE signaling prevents IgE-memory formation. Nat Immunol (2016) 17:1109–17. doi: 10.1038/ni.3508
58. Lens SM, Keehnen RM, van Oers MH, van Lier RA, Pals ST, Koopman G. Identification of a novel subpopulation of germinal center B cells characterized by expression of IgD and CD70. Eur J Immunol (1996) 26:1007–11. doi: 10.1002/eji.1830260508
59. Kobata T, Jacquot S, Kozlowski S, Agematsu K, Schlossman SF, Morimoto C. CD27-CD70 interactions regulate B-cell activation by T cells. Proc Natl Acad Sci USA (1995) 92:11249–53. doi: 10.1073/pnas.92.24.11249
60. Seifert M, Küppers R. Molecular footprints of a germinal center derivation of human IgM+(IgD+)CD27+ B cells and the dynamics of memory B cell generation. J Exp Med (2009) 206:2659–69. doi: 10.1084/jem.20091087
61. Aranburu A, Piano Mortari E, Baban A, Giorda E, Cascioli S, Marcellini V, et al. Human B-cell memory is shaped by age- and tissue-specific T-independent and GC-dependent events. Eur J Immunol (2017) 47:327–44. doi: 10.1002/eji.201646642
62. Sanz I, Wei C, Lee FE, Anolik J. Phenotypic and functional heterogeneity of human memory B cells. Semin Immunol (2008) 20:67–82. doi: 10.1016/j.smim.2007.12.006
63. van Gent R, van Tilburg CM, Nibbelke EE, Otto SA, Gaiser JF, Janssens-Korpela PL, et al. Refined characterization and reference values of the pediatric T- and B-cell compartments. Clin Immunol (2009) 133:95–107. doi: 10.1016/j.clim.2009.05.020
64. Kibler A, Budeus B, Küppers R, Seifert M. The splenic marginal zone in children Is characterized by a subpopulation of CD27-negative, lowly IGHV-mutated B cells. Front Immunol (2022) 13:825619. doi: 10.3389/fimmu.2022.825619
65. Grimsholm O, Piano Mortari E, Davydov AN, Shugay M, Obraztsova AS, Bocci C, et al. The interplay between CD27(dull) and CD27(bright) B cells ensures the flexibility, stability, and resilience of human B cell memory. Cell Rep (2020) 30:2963–77 e6. doi: 10.1016/j.celrep.2020.02.022
66. Bianchi A, Scherer M, Zaurin R, Quililan K, Velten L, Beekman R. scTAM-seq enables targeted high-confidence analysis of DNA methylation in single cells. Genome Biol (2022) 23:229. doi: 10.1186/s13059-022-02796-7
67. Moir S, Malaspina A, Ogwaro KM, Donoghue ET, Hallahan CW, Ehler LA, et al. HIV-1 induces phenotypic and functional perturbations of B cells in chronically infected individuals. Proc Natl Acad Sci USA (2001) 98:10362–7. doi: 10.1073/pnas.181347898
68. Warnatz K, Wehr C, Drager R, Schmidt S, Eibel H, Schlesier M, et al. Expansion of CD19(hi)CD21(lo/neg) B cells in common variable immunodeficiency (CVID) patients with autoimmune cytopenia. Immunobiology (2002) 206:502–13. doi: 10.1078/0171-2985-00198
69. Gjertsson I, McGrath S, Grimstad K, Jonsson CA, Camponeschi A, Thorarinsdottir K, et al. A close-up on the expanding landscape of CD21-/low B cells in humans. Clin Exp Immunol (2022) 210:217–29. doi: 10.1093/cei/uxac103
70. Keller B, Warnatz K. T-bet(high)CD21(low) B cells: the need to unify our understanding of a distinct B cell population in health and disease. Curr Opin Immunol (2023) 82:102300. doi: 10.1016/j.coi.2023.102300
71. Cancro MP. Age-associated B cells. Annu Rev Immunol (2020) 38:315–40. doi: 10.1146/annurev-immunol-092419-031130
72. Szabo SJ, Kim ST, Costa GL, Zhang X, Fathman CG, Glimcher LH. A novel transcription factor, T-bet, directs Th1 lineage commitment. Cell (2000) 100:655–69. doi: 10.1016/s0092-8674(00)80702-3
73. Johnson JL, Rosenthal RL, Knox JJ, Myles A, Naradikian MS, Madej J, et al. The transcription factor T-bet resolves memory B cell subsets with distinct tissue distributions and antibody specificities in mice and humans. Immunity (2020) 52:842–55 e6. doi: 10.1016/j.immuni.2020.03.020
74. Knox JJ, Buggert M, Kardava L, Seaton KE, Eller MA, Canaday DH, et al. T-bet+ B cells are induced by human viral infections and dominate the HIV gp140 response. JCI Insight (2017) 2:e92943. doi: 10.1172/jci.insight.92943
75. Weller S, Faili A, Garcia C, Braun MC, Le Deist FF, de Saint Basile GG, et al. CD40-CD40L independent Ig gene hypermutation suggests a second B cell diversification pathway in humans. Proc Natl Acad Sci USA (2001) 98:1166–70. doi: 10.1073/pnas.98.3.1166
76. Weller S, Mamani-Matsuda M, Picard C, Cordier C, Lecoeuche D, Gauthier F, et al. Somatic diversification in the absence of antigen-driven responses is the hallmark of the IgM+ IgD+ CD27+ B cell repertoire in infants. J Exp Med (2008) 205:1331–42. doi: 10.1084/jem.20071555
77. Reynaud CA, Garcia C, Hein WR, Weill JC. Hypermutation generating the sheep immunoglobulin repertoire is an antigen-independent process. Cell (1995) 80:115–25. doi: 10.1016/0092-8674(95)90456-5
78. Scheeren FA, Nagasawa M, Weijer K, Cupedo T, Kirberg J, Legrand N, et al. T cell-independent development and induction of somatic hypermutation in human IgM+ IgD+ CD27+ B cells. J Exp Med (2008) 205:2033–42. doi: 10.1084/jem.20070447
79. Facchetti F, Appiani C, Salvi L, Levy J, Notarangelo LD. Immunohistologic analysis of ineffective CD40-CD40 ligand interaction in lymphoid tissues from patients with X-linked immunodeficiency with hyper-IgM. Abortive germinal center cell reaction and severe depletion of follicular dendritic cells. J Immunol (1995) 154:6624–33. doi: 10.4049/jimmunol.154.12.6624
80. Brodeur SR, Angelini F, Bacharier LB, Blom AM, Mizoguchi E, Fujiwara H, et al. C4b-binding protein (C4BP) activates B cells through the CD40 receptor. Immunity (2003) 18:837–48. doi: 10.1016/s1074-7613(03)00149-3
81. Kuraoka M, Liao D, Yang K, Allgood SD, Levesque MC, Kelsoe G, et al. Activation-induced cytidine deaminase expression and activity in the absence of germinal centers: insights into hyper-IgM syndrome. J Immunol (2009) 183:3237–48. doi: 10.4049/jimmunol.0901548
82. Aranburu A, Ceccarelli S, Giorda E, Lasorella R, Ballatore G, Carsetti R. TLR ligation triggers somatic hypermutation in transitional B cells inducing the generation of IgM memory B cells. J Immunol (2010) 185:7293–301. doi: 10.4049/jimmunol.1002722
83. Cantaert T, Schickel JN, Bannock JM, Ng YS, Massad C, Oe T, et al. Activation-induced cytidine deaminase expression in human B cell precursors Is essential for central B cell tolerance. Immunity (2015) 43:884–95. doi: 10.1016/j.immuni.2015.10.002
84. Kruetzmann S, Rosado MM, Weber H, Germing U, Tournilhac O, Peter HH, et al. Human immunoglobulin M memory B cells controlling Streptococcus pneumoniae infections are generated in the spleen. J Exp Med (2003) 197:939–45. doi: 10.1084/jem.20022020
85. Weller S, Braun MC, Tan BK, Rosenwald A, Cordier C, Conley ME, et al. Human blood IgM "memory" B cells are circulating splenic marginal zone B cells harboring a prediversified immunoglobulin repertoire. Blood (2004) 104:3647–54. doi: 10.1182/blood-2004-01-0346
86. Cameron PU, Jones P, Gorniak M, Dunster K, Paul E, Lewin S, et al. Splenectomy associated changes in IgM memory B cells in an adult spleen registry cohort. PloS One (2011) 6:e23164. doi: 10.1371/journal.pone.0023164
87. Wasserstrom H, Bussel J, Lim LC, Cunningham-Rundles C. Memory B cells and pneumococcal antibody after splenectomy. J Immunol (2008) 181:3684–9. doi: 10.4049/jimmunol.181.5.3684
88. Mamani-Matsuda M, Cosma A, Weller S, Faili A, Staib C, Garcon L, et al. The human spleen is a major reservoir for long-lived vaccinia virus-specific memory B cells. Blood (2008) 111:4653–9. doi: 10.1182/blood-2007-11-123844
89. Cattoretti G, Buttner M, Shaknovich R, Kremmer E, Alobeid B, Niedobitek G. Nuclear and cytoplasmic AID in extrafollicular and germinal center B cells. Blood (2006) 107:3967–75. doi: 10.1182/blood-2005-10-4170
90. Steiniger BS, Raimer L, Ecke A, Stuck BA, Cetin Y. Plasma cells, plasmablasts, and AID(+)/CD30(+) B lymphoblasts inside and outside germinal centres: details of the basal light zone and the outer zone in human palatine tonsils. Histochem Cell Biol (2020) 154:55–75. doi: 10.1007/s00418-020-01861-1
91. Willenbrock K, Jungnickel B, Hansmann ML, Küppers R. Human splenic marginal zone B cells lack expression of activation-induced cytidine deaminase. Eur J Immunol (2005) 35:3002–7. doi: 10.1002/eji.200535134
92. Liu X, Zhao Y, Qi H. T-independent antigen induces humoral memory through germinal centers. J Exp Med (2022) 219:e20210527. doi: 10.1084/jem.20210527
93. de Vinuesa CG, Cook MC, Ball J, Drew M, Sunners Y, Cascalho M, et al. Germinal centers without T cells. J Exp Med (2000) 191:485–94. doi: 10.1084/jem.191.3.485
94. Weller S, Sterlin D, Fadeev T, Coignard E, Verge de Los Aires A, Goetz C, et al. T-independent responses to polysaccharides in humans mobilize marginal zone B cells prediversified against gut bacterial antigens. Sci Immunol (2023) 8:eade1413. doi: 10.1126/sciimmunol.ade1413
95. Moens L, Wuyts M, Meyts I, De Boeck K, Bossuyt X. Human memory B lymphocyte subsets fulfill distinct roles in the anti-polysaccharide and anti-protein immune response. J Immunol (2008) 181:5306–12. doi: 10.4049/jimmunol.181.8.5306
96. Hougs L, Juul L, Ditzel HJ, Heilmann C, Svejgaard A, Barington T. The first dose of a Haemophilus influenzae type b conjugate vaccine reactivates memory B cells: evidence for extensive clonal selection, intraclonal affinity maturation, and multiple isotype switches to IgA2. J Immunol (1999) 162:224–37. doi: 10.4049/jimmunol.162.1.224
97. Warnatz K, Bossaller L, Salzer U, Skrabl-Baumgartner A, Schwinger W, van der Burg M, et al. Human ICOS deficiency abrogates the germinal center reaction and provides a monogenic model for common variable immunodeficiency. Blood (2006) 107:3045–52. doi: 10.1182/blood-2005-07-2955
98. McAdam AJ, Greenwald RJ, Levin MA, Chernova T, Malenkovich N, Ling V, et al. ICOS is critical for CD40-mediated antibody class switching. Nature (2001) 409:102–5. doi: 10.1038/35051107
99. Klein U, Küppers R, Rajewsky K. Evidence for a large compartment of IgM-expressing memory B cells in humans. Blood (1997) 89:1288–98. doi: 10.1182/blood.V89.4.1288
100. Kibler A, Budeus B, Homp E, Bronischewski K, Berg V, Sellmann L, et al. Systematic memory B cell archiving and random display shape the human splenic marginal zone throughout life. J Exp Med (2021) 218:e20201952. doi: 10.1084/jem.20201952
101. Richards SJ, Morgan GJ, Hillmen P. Immunophenotypic analysis of B cells in PNH: insights into the generation of circulating naive and memory B cells. Blood (2000) 96:3522–8. doi: 10.1182/blood.V96.10.3522
102. Ciabattini A, Pastore G, Lucchesi S, Montesi G, Costagli S, Polvere J, et al. Trajectory of spike-specific B cells elicited by two doses of BNT162b2 mRNA vaccine. Cells (2023) 12(13):1706. doi: 10.3390/cells12131706
103. Phad GE, Pinto D, Foglierini M, Akhmedov M, Rossi RL, Malvicini E, et al. Clonal structure, stability and dynamics of human memory B cells and circulating plasmablasts. Nat Immunol (2022) 23:1076–85. doi: 10.1038/s41590-022-01230-1
104. Mikelov A, Alekseeva EI, Komech EA, Staroverov DB, Turchaninova MA, Shugay M, et al. Memory persistence and differentiation into antibody-secreting cells accompanied by positive selection in longitudinal BCR repertoires. Elife (2022) 11:e79254. doi: 10.7554/eLife.79254
105. Dan JM, Mateus J, Kato Y, Hastie KM, Yu ED, Faliti CE, et al. Immunological memory to SARS-CoV-2 assessed for up to 8 months after infection. Science (2021) 371:eabf4063. doi: 10.1126/science.abf4063
106. Koethe S, Zander L, Köster S, Annan A, Ebenfelt A, Spencer J, et al. Pivotal advance: CD45RB glycosylation is specifically regulated during human peripheral B cell differentiation. J Leukoc Biol (2011) 90:5–19. doi: 10.1189/jlb.0710404
107. Veneri D, Ortolani R, Franchini M, Tridente G, Pizzolo G, Vella A. Expression of CD27 and CD23 on peripheral blood B lymphocytes in humans of different ages. Blood Transfus (2009) 7:29–34. doi: 10.2450/2008.0007-08
108. Oakes CC, Seifert M, Assenov Y, Gu L, Przekopowitz M, Ruppert AS, et al. DNA methylation dynamics during B cell maturation underlie a continuum of disease phenotypes in chronic lymphocytic leukemia. Nat Genet (2016) 48:253–64. doi: 10.1038/ng.3488
109. Dogan I, Bertocci B, Vilmont V, Delbos F, Megret J, Storck S, et al. Multiple layers of B cell memory with different effector functions. Nat Immunol (2009) 10:1292–9. doi: 10.1038/ni.1814
110. Pape KA, Taylor JJ, Maul RW, Gearhart PJ, Jenkins MK. Different B cell populations mediate early and late memory during an endogenous immune response. Science (2011) 331:1203–7. doi: 10.1126/science.1201730
111. Pasqualucci L, Migliazza A, Fracchiolla N, William C, Neri A, Baldini L, et al. BCL-6 mutations in normal germinal center B cells: evidence of somatic hypermutation acting outside Ig loci. Proc Natl Acad Sci USA (1998) 95:11816–21. doi: 10.1073/pnas.95.20.11816
112. Fukita Y, Jacobs H, Rajewsky K. Somatic hypermutation in the heavy chain locus correlates with transcription. Immunity (1998) 9:105–14. doi: 10.1016/s1074-7613(00)80592-0
113. Bachl J, Carlson C, Gray-Schopfer V, Dessing M, Olsson C. Increased transcription levels induce higher mutation rates in a hypermutating cell line. J Immunol (2001) 166:5051–7. doi: 10.4049/jimmunol.166.8.5051
114. Yang SY, Fugmann SD, Schatz DG. Control of gene conversion and somatic hypermutation by immunoglobulin promoter and enhancer sequences. J Exp Med (2006) 203:2919–28. doi: 10.1084/jem.20061835
115. Ye BH, Lista F, Lo Coco F, Knowles DM, Offit K, Chaganti RS, et al. Alterations of a zinc finger-encoding gene, BCL-6, in diffuse large-cell lymphoma. Science (1993) 262:747–50. doi: 10.1126/science.8235596
116. Klein U, Dalla-Favera R. Germinal centres: role in B-cell physiology and Malignancy. Nat Rev Immunol (2008) 8:22–33. doi: 10.1038/nri2217
117. Bagnara D, Squillario M, Kipling D, Mora T, Walczak AM, Da Silva L, et al. A reassessment of IgM memory subsets in humans. J Immunol (2015) 195:3716–24. doi: 10.4049/jimmunol.1500753
118. Ota M, Nakano M, Nagafuchi Y, Kobayashi S, Hatano H, Yoshida R, et al. Multimodal repertoire analysis unveils B cell biology in immune-mediated diseases. Ann Rheum Dis (2023) 82:1455–63. doi: 10.1136/ard-2023-224421
119. Ghraichy M, von Niederhäusern V, Kovaltsuk A, Galson JD, Deane CM, Trück J. Different B cell subpopulations show distinct patterns in their IgH repertoire metrics. Elife (2021) 10:e73111. doi: 10.7554/eLife.73111
120. Pugh-Bernard AE, Silverman GJ, Cappione AJ, Villano ME, Ryan DH, Insel RA, et al. Regulation of inherently autoreactive VH4-34 B cells in the maintenance of human B cell tolerance. J Clin Invest (2001) 108:1061–70. doi: 10.1172/JCI12462
121. Wardemann H, Yurasov S, Schaefer A, Young JW, Meffre E, Nussenzweig MC. Predominant autoantibody production by early human B cell precursors. Science (2003) 301:1374–7. doi: 10.1126/science.1086907
122. Horns F, Vollmers C, Croote D, Mackey SF, Swan GE, Dekker CL, et al. Lineage tracing of human B cells reveals the in vivo landscape of human antibody class switching. Elife (2016) 5:e16578. doi: 10.7554/eLife.16578
123. Zhao Y, Uduman M, Siu JHY, Tull TJ, Sanderson JD, Wu YB, et al. Spatiotemporal segregation of human marginal zone and memory B cell populations in lymphoid tissue. Nat Commun (2018) 9:3857. doi: 10.1038/s41467-018-06089-1
124. Narvaez CF, Feng N, Vasquez C, Sen A, Angel J, Greenberg HB, et al. Human rotavirus-specific IgM Memory B cells have differential cloning efficiencies and switch capacities and play a role in antiviral immunity in vivo. J Virol (2012) 86:10829–40. doi: 10.1128/JVI.01466-12
125. Tian C, Luskin GK, Dischert KM, Higginbotham JN, Shepherd BE, Crowe JE Jr. Immunodominance of the VH1-46 antibody gene segment in the primary repertoire of human rotavirus-specific B cells is reduced in the memory compartment through somatic mutation of nondominant clones. J Immunol (2008) 180:3279–88. doi: 10.4049/jimmunol.180.5.3279
126. Tuaillon E, Tabaa YA, Petitjean G, Huguet MF, Pajeaux G, Fondere JM, et al. Detection of memory B lymphocytes specific to hepatitis B virus (HBV) surface antigen (HBsAg) from HBsAg-vaccinated or HBV-immunized subjects by ELISPOT assay. J Immunol Methods (2006) 315:144–52. doi: 10.1016/j.jim.2006.07.016
127. Scherer EM, Smith RA, Simonich CA, Niyonzima N, Carter JJ, Galloway DA. Characteristics of memory B cells elicited by a highly efficacious HPV vaccine in subjects with no pre-existing immunity. PloS Pathog (2014) 10:e1004461. doi: 10.1371/journal.ppat.1004461
128. Lindner JM, Cornacchione V, Sathe A, Be C, Srinivas H, Riquet E, et al. Human memory B cells harbor diverse cross-neutralizing antibodies against BK and JC polyomaviruses. Immunity (2019) 50:668–76 e5. doi: 10.1016/j.immuni.2019.02.003
129. Della Valle L, Dohmen SE, Verhagen OJ, Berkowska MA, Vidarsson G, Ellen van der Schoot C. The majority of human memory B cells recognizing RhD and tetanus resides in IgM+ B cells. J Immunol (2014) 193:1071–9. doi: 10.4049/jimmunol.1400706
130. Tjiam MC, Fernandez S, French MA. Characterising the phenotypic diversity of antigen-specific memory B cells before and after vaccination. Front Immunol (2021) 12:738123. doi: 10.3389/fimmu.2021.738123
131. Hopp CS, Sekar P, Diouf A, Miura K, Boswell K, Skinner J, et al. Plasmodium falciparum-specific IgM B cells dominate in children, expand with malaria, and produce functional IgM. J Exp Med (2021) 218:e20200901. doi: 10.1084/jem.20200901
132. Cesta MF. Normal structure, function, and histology of the spleen. Toxicol Pathol (2006) 34:455–65. doi: 10.1080/01926230600867743
133. Kraal G. Cells in the marginal zone of the spleen. Int Rev Cytol (1992) 132:31–74. doi: 10.1016/s0074-7696(08)62453-5
134. Kibler A, Seifert M, Budeus B. Age-related changes of the human splenic marginal zone B cell compartment. Immunol Lett (2023) 256-257:59–65. doi: 10.1016/j.imlet.2023.04.003
135. Cerutti A, Cols M, Puga I. Marginal zone B cells: virtues of innate-like antibody-producing lymphocytes. Nat Rev Immunol (2013) 13:118–32. doi: 10.1038/nri3383
136. Timens W, Poppema S. Lymphocyte compartments in human spleen. An immunohistologic study in normal spleens and uninvolved spleens in Hodgkin's disease. Am J Pathol (1985) 120:443–54.
137. Puga I, Cols M, Barra CM, He B, Cassis L, Gentile M, et al. B cell-helper neutrophils stimulate the diversification and production of immunoglobulin in the marginal zone of the spleen. Nat Immunol (2012) 13:170–80. doi: 10.1038/ni.2194
138. Steiniger B, Timphus EM, Jacob R, Barth PJ. CD27+ B cells in human lymphatic organs: re-evaluating the splenic marginal zone. Immunology (2005) 116:429–42. doi: 10.1111/j.1365-2567.2005.02242.x
139. Magri G, Miyajima M, Bascones S, Mortha A, Puga I, Cassis L, et al. Innate lymphoid cells integrate stromal and immunological signals to enhance antibody production by splenic marginal zone B cells. Nat Immunol (2014) 15:354–64. doi: 10.1038/ni.2830
140. Steiniger B, Barth P, Hellinger A. The perifollicular and marginal zones of the human splenic white pulp : do fibroblasts guide lymphocyte immigration? Am J Pathol (2001) 159:501–12. doi: 10.1016/S0002-9440(10)61722-1
141. Steiniger B, Trabandt M, Barth PJ. The follicular dendritic cell network in secondary follicles of human palatine tonsils and spleens. Histochem Cell Biol (2011) 135:327–36. doi: 10.1007/s00418-011-0799-x
142. Steiniger BS, Wilhelmi V, Seiler A, Lampp K, Stachniss V. Heterogeneity of stromal cells in the human splenic white pulp. Fibroblastic reticulum cells, follicular dendritic cells and a third superficial stromal cell type. Immunology (2014) 143:462–77. doi: 10.1111/imm.12325
143. Chorny A, Casas-Recasens S, Sintes J, Shan M, Polentarutti N, Garcia-Escudero R, et al. The soluble pattern recognition receptor PTX3 links humoral innate and adaptive immune responses by helping marginal zone B cells. J Exp Med (2016) 213:2167–85. doi: 10.1084/jem.20150282
144. Sintes J, Gentile M, Zhang S, Garcia-Carmona Y, Magri G, Cassis L, et al. mTOR intersects antibody-inducing signals from TACI in marginal zone B cells. Nat Commun (2017) 8:1462. doi: 10.1038/s41467-017-01602-4
145. Maglione PJ, Simchoni N, Black S, Radigan L, Overbey JR, Bagiella E, et al. IRAK-4 and MyD88 deficiencies impair IgM responses against T-independent bacterial antigens. Blood (2014) 124:3561–71. doi: 10.1182/blood-2014-07-587824
146. Tull TJ, Pitcher MJ, Guesdon W, Siu JHY, Lebrero-Fernandez C, Zhao Y, et al. Human marginal zone B cell development from early T2 progenitors. J Exp Med (2021) 218:e20202001. doi: 10.1084/jem.20202001
147. Siu JHY, Pitcher MJ, Tull TJ, Velounias RL, Guesdon W, Montorsi L, et al. Two subsets of human marginal zone B cells resolved by global analysis of lymphoid tissues and blood. Sci Immunol (2022) 7:eabm9060. doi: 10.1126/sciimmunol.abm9060
148. Haas A, Zimmermann K, Graw F, Slack E, Rusert P, Ledergerber B, et al. Systemic antibody responses to gut commensal bacteria during chronic HIV-1 infection. Gut (2011) 60:1506–19. doi: 10.1136/gut.2010.224774
149. Weller S, Bonnet M, Delagreverie H, Israel L, Chrabieh M, Marodi L, et al. IgM+IgD+CD27+ B cells are markedly reduced in IRAK-4-, MyD88-, and TIRAP- but not UNC-93B-deficient patients. Blood (2012) 120:4992–5001. doi: 10.1182/blood-2012-07-440776
150. Descatoire M, Weller S, Irtan S, Sarnacki S, Feuillard J, Storck S, et al. Identification of a human splenic marginal zone B cell precursor with NOTCH2-dependent differentiation properties. J Exp Med (2014) 211:987–1000. doi: 10.1084/jem.20132203
151. Glass DR, Tsai AG, Oliveria JP, Hartmann FJ, Kimmey SC, Calderon AA, et al. An integrated multi-omic single-cell atlas of human B cell identity. Immunity (2020) 53:217–32 e5. doi: 10.1016/j.immuni.2020.06.013
152. Steiniger B, Barth P, Herbst B, Hartnell A, Crocker PR. The species-specific structure of microanatomical compartments in the human spleen: strongly sialoadhesin-positive macrophages occur in the perifollicular zone, but not in the marginal zone. Immunology (1997) 92:307–16. doi: 10.1046/j.1365-2567.1997.00328.x
153. Tangye SG, Liu YJ, Aversa G, Phillips JH, de Vries JE. Identification of functional human splenic memory B cells by expression of CD148 and CD27. J Exp Med (1998) 188:1691–703. doi: 10.1084/jem.188.9.1691
154. Saito T, Chiba S, Ichikawa M, Kunisato A, Asai T, Shimizu K, et al. Notch2 is preferentially expressed in mature B cells and indispensable for marginal zone B lineage development. Immunity (2003) 18:675–85. doi: 10.1016/s1074-7613(03)00111-0
155. Steiniger B, Timphus EM, Barth PJ. The splenic marginal zone in humans and rodents: an enigmatic compartment and its inhabitants. Histochem Cell Biol (2006) 126:641–8. doi: 10.1007/s00418-006-0210-5
156. Dunn-Walters DK, Isaacson PG, Spencer J. Analysis of mutations in immunoglobulin heavy chain variable region genes of microdissected marginal zone (MGZ) B cells suggests that the MGZ of human spleen is a reservoir of memory B cells. J Exp Med (1995) 182:559–66. doi: 10.1084/jem.182.2.559
157. Tangye SG, Good KL. Human IgM+CD27+ B cells: memory B cells or "memory" B cells? J Immunol (2007) 179:13–9. doi: 10.4049/jimmunol.179.1.13
158. Steiniger BS. Human spleen microanatomy: why mice do not suffice. Immunology (2015) 145:334–46. doi: 10.1111/imm.12469
159. Dammers PM, Visser A, Popa ER, Nieuwenhuis P, Kroese FG. Most marginal zone B cells in rat express germline encoded Ig VH genes and are ligand selected. J Immunol (2000) 165:6156–69. doi: 10.4049/jimmunol.165.11.6156
160. Anderson SM, Tomayko MM, Ahuja A, Haberman AM, Shlomchik MJ. New markers for murine memory B cells that define mutated and unmutated subsets. J Exp Med (2007) 204:2103–14. doi: 10.1084/jem.20062571
161. Mateo MS, Mollejo M, Villuendas R, Algara P, Sanchez-Beato M, Martinez P, et al. Molecular heterogeneity of splenic marginal zone lymphomas: analysis of mutations in the 5' non-coding region of the bcl-6 gene. Leukemia (2001) 15:628–34. doi: 10.1038/sj.leu.2402073
162. Giesecke C, Frölich D, Reiter K, Mei HE, Wirries I, Kuhly R, et al. Tissue distribution and dependence of responsiveness of human antigen-specific memory B cells. J Immunol (2014) 192:3091–100. doi: 10.4049/jimmunol.1302783
163. Mandric I, Rotman J, Yang HT, Strauli N, Montoya DJ, van der Wey W, et al. Profiling immunoglobulin repertoires across multiple human tissues using RNA sequencing. Nat Commun (2020) 11:3126. doi: 10.1038/s41467-020-16857-7
164. Meng W, Zhang B, Schwartz GW, Rosenfeld AM, Ren D, Thome JJC, et al. An atlas of B-cell clonal distribution in the human body. Nat Biotechnol (2017) 35:879–84. doi: 10.1038/nbt.3942
165. Mond JJ, Lees A, Snapper CM. T cell-independent antigens type 2. Annu Rev Immunol (1995) 13:655–92. doi: 10.1146/annurev.iy.13.040195.003255
166. Siegrist CA, Aspinall R. B-cell responses to vaccination at the extremes of age. Nat Rev Immunol (2009) 9:185–94. doi: 10.1038/nri2508
167. Goronzy JJ, Gustafson CE, Weyand CM. Immune deficiencies at the extremes of age. In: Rich RR, Fleisher TA, Shearer WT, Schroeder HW, Frw AJ, Weyand CM, editors. Clinical Immunology. Amsterdam, Netherlands: Elsevier (2019). p. 535–43.e1.
168. Zandvoort A, Lodewijk ME, de Boer NK, Dammers PM, Kroese FG, Timens W. CD27 expression in the human splenic marginal zone: the infant marginal zone is populated by naive B cells. Tissue Antigens (2001) 58:234–42. doi: 10.1034/j.1399-0039.2001.580403.x
169. Timens W, Boes A, Rozeboom-Uiterwijk T, Poppema S. Immaturity of the human splenic marginal zone in infancy. Possible contribution to the deficient infant immune response. J Immunol (1989) 143:3200–6. doi: 10.4049/jimmunol.143.10.3200
Keywords: B cells, CD27, class switch recombination, germinal center, IgD, immunoglobulin V genes, marginal zone, somatic hypermutation
Citation: Budeus B, Kibler A and Küppers R (2023) Human IgM–expressing memory B cells. Front. Immunol. 14:1308378. doi: 10.3389/fimmu.2023.1308378
Received: 06 October 2023; Accepted: 27 November 2023;
Published: 08 December 2023.
Edited by:
Takeshi Inoue, The University of Tokyo, JapanReviewed by:
Christopher Sundling, Karolinska Institutet (KI), SwedenTomoharu Yasuda, Hiroshima University, Japan
Copyright © 2023 Budeus, Kibler and Küppers. This is an open-access article distributed under the terms of the Creative Commons Attribution License (CC BY). The use, distribution or reproduction in other forums is permitted, provided the original author(s) and the copyright owner(s) are credited and that the original publication in this journal is cited, in accordance with accepted academic practice. No use, distribution or reproduction is permitted which does not comply with these terms.
*Correspondence: Ralf Küppers, ralf.kueppers@uk-essen.de