Resource diversity mitigates the effects of intraspecific competition in co-occurring cryptic nematode species
- 1Marine Biology Unit, Department of Biology, Ghent University, Ghent, Belgium
- 2Flanders Research Institute for Agriculture, Fisheries and Food (ILVO), Aquatic Environment and Quality, Oostende, Belgium
Intraspecific competition and resource diversity are considered major drivers of niche differentiation, which are expected to promote population niche expansion by driving individuals to feed on alternative resources and/or by enhancing individual diet specialization. Nevertheless, experimental studies on the interaction effects of both factors on animal behavior and population dynamics remain scant. Here, we investigate how resource diversity alters the impact of intraspecific competition on resource preference and fitness of three co-occurring cryptic species of the marine nematode complex Litoditis marina (Pm I, Pm III and Pm IV). For each cryptic species, two competition regimes (‘low nematode density’ and ‘high nematode density’) were established in microcosms with varying resource diversity (E. coli, low-, medium- and high-diversity food). Our results show differences in resource preference and population fitness depending on intraspecific competition and resource diversity, but the response also varied considerably between cryptic species. Pm III did not exhibit resource preference under low intraspecific competition, but preferred the two most diverse food sources under high intraspecific competition. Pm IV also showed preference for medium-diversity food under high competition, whereas no resource preference was observed in Pm I regardless of competition regimes and resource diversity. Nevertheless, all cryptic species exhibited enhanced adult population growth on a more diverse food source under stronger intraspecific competition. These results indicate that resource diversity can alleviate intraspecific competition and affect niche diversification, which may impact diversity maintenance in ecological communities.
1 Introduction
Intraspecific competition and ecological opportunity are considered major drivers of population and individual niche differentiation (Van Valen, 1965; Araújo et al., 2011), which can have significant ramifications on ecological diversification within populations (Schluter, 2000), on ecosystem functioning (Vrede et al., 2011), and on diversity maintenance in biological communities (Violle et al., 2012; Hart et al., 2016; Hausch et al., 2018). Intraspecific competition is expected to drive niche expansion because resource scarcity due to strong competition will force individuals to feed on previously unutilized and/or suboptimal resources (MacArthur and Levins, 1964; Pulliam, 1974; Stephens and Krebs, 1986); such prediction is supported by a wide variety of empirical studies (Araújo et al., 2011). A growing number of studies also revealed that ecological opportunity, such as resource diversity, can lead to niche expansion through enhanced individual specialization (Evangelista et al., 2014; Costa-Pereira et al., 2017; Bolnick and Ballare, 2020). Despite compelling evidence that intraspecific competition and resource diversity promote niche expansion, experimental studies on how the interaction of both factors alters trophic niches and population dynamics remain scant.
The trophic niche width of a population, which is determined by individual diet width (‘within-individual component’) and among-individual diet width (‘between-individual component’) (Roughgarden, 1972), expands when individuals add new resources to their diet and thus become more generalists (i.e., increase in individual diet width), or when individuals reduce diet overlap by feeding on different resources and thus remain relatively specialized (i.e., increase in among-individual diet width) (Araújo et al., 2011). Population niche expansion can result in niche diversification if population niche width increases mainly through among-individual diet variation (Bolnick et al., 2003; Svanbäck and Bolnick, 2005; Bolnick et al., 2007). This ‘among-individual diet variation’ – in which individuals use different subsets of the population’s dietary resources – is generally explained by Optimal Foraging Theory (OFT) (Stephens and Krebs, 1986). This theory suggests that individuals act to maximize their rate of energy intake by feeding on the most valuable resources and ignoring lower-value resources when search and handling time could be more profitably spent foraging for more valuable ones. When preferred resources are limited, individuals will expand their niche to add new resource types to their diet. Empirical studies show that intraspecific competition and resource diversity can expand population niche width by promoting among-individual diet variation (Araújo et al., 2011), favouring diet shift via phenotypic plasticity or behavioral changes (Svanbäck and Bolnick, 2007; Svanbäck et al., 2009; Meyer et al., 2016; Adey and Larson, 2021).
But why would individuals within the same population consume different resources? Araújo et al. (2011) suggested three scenarios that can lead to among-individual diet variation: individuals may have different optimal diets because: 1) they do not have the same resource rank preferences, reflecting among-individual variation in searching, recognizing, and handling alternative resources; 2) they utilize different optimization criteria and/or differ in fitness and physiological requirements; and 3) they have different competitive abilities and/or feeding strategies that influence how they obtain their preferred food. Thus, the effects of intraspecific competition and resource diversity on trophic niches will largely depend on how these factors influence the foraging behavior and fitness of organisms (Bolnick et al., 2003; Araújo et al., 2011).
While it is widely accepted that intraspecific competition can have adverse effects on life-history characteristics of organisms (Fowler, 1987; Brook and Bradshaw, 2006; Lamb et al., 2017; Evangelista et al., 2020), the question whether resource diversity has positive or negative effects remains a matter for debate (Duffy, 2002). Some theories predict that diverse food sources will lower the fitness of consumers since these will have higher probability of containing food items that are resistant to consumption (‘variance-in-edibility hypothesis’) (Leibold, 1989; Hillebrand and Shurin, 2005), or will have reduced abundances of preferred food available for the consumers, which may decrease their foraging efficiency (‘dilution or resource concentration hypothesis’) (Andow, 1991; Joshi et al., 2004). On the other hand, a diverse food source may be beneficial for consumers as it can provide a more complete range of nutritional resources (‘balanced-diet hypothesis’) (DeMott, 1998). The increase in resource diversity may also be particularly important in the light of increasing anthropogenic disturbances (e.g., climate change and pollution) as it can potentially increase the chances of survival of consumers when certain resources disappear or become depleted (Birnie-Gauvin et al., 2017). Therefore, although resource diversity can mitigate the negative effects of intraspecific competition through enhanced individual diet specialization (see above), the outcome may depend on how animals respond to resource diversity.
In the present study, we used the cryptic nematode species complex Litoditis marina (Sudhaus, 2011) to investigate the interaction effects of intraspecific competition (conspecific density) and resource (bacteria) diversity on foraging behavior and population fitness of organisms. Litoditis marina is a bacterial-feeding cryptic nematode species complex associated with living and decomposing macroalgae in intertidal zones, and consists of species that often co-occur at local scales (Derycke et al., 2006, Derycke et al., 2008). These co-occurring species have partly different ecological and functional traits (De Meester et al., 2011, De Meester et al., 2012; De Meester et al., 2015a, b, De Meester et al., 2016), exhibit subtle differences in microhabitat preferences (Guden et al., 2018), and respond differently to gradients of resource diversity (Guden et al., 2021), indicating niche differentiation between cryptic species. In addition, intraspecific competition is prominent in L. marina (De Meester et al., 2015a), with pronounced intraspecific microbiome variability that – among other things – may indicate individual dietary specialization (Derycke et al., 2016; Vafeiadou et al., 2022). Nevertheless, whether food diversity can alter the outcome of intraspecific competition in these co-occurring cryptic species remains to be tested.
Here, we investigated food preference (based on taxis-to-food assays) and fitness (minimum juvenile development time, total fecundity and adult population growth) of three cryptic species (Pm I, Pm III and Pm IV) of L. marina under different intraspecific-competition regimes (low and high nematode density) and with different food-diversity treatments (E. coli, low-, medium-, and high-diversity food). We hypothesized that: 1) all cryptic species would perform less well under high intraspecific competition than under low competition regardless of food diversity; 2) the preference and fitness of all cryptic species would not differ between food-diversity treatments under low intraspecific competition because there is less competition for resources; 3) all cryptic species would exhibit preference for a more diverse resource under high intraspecific competition, minimizing competition through population trophic niche expansion; and 4) all cryptic species would exhibit higher relative fitness on a more diverse resource under high intraspecific competition as predicted by the balanced-diet hypothesis. Investigating how food diversity influences intraspecific competition in cryptic species may deepen our understanding on the mechanisms that affect population and community dynamics, and how diversity is maintained in ecological communities.
2 Materials and methods
2.1 Nematode stock cultures
Nematodes of Litoditis marina used for the experiments were harvested from stock cultures in exponential growth phase. Monospecific cultures of three cryptic species (Pm I, Pm III, and Pm IV) were raised from single gravid females obtained from the field. Pm I and Pm III originated from the Paulina salt marsh, Schelde Estuary, The Netherlands (51° 21’ N, 3° 49’ E), whereas Pm IV was originally isolated from Lake Grevelingen, a brackish-water lake in The Netherlands (51° 44’ N, 3° 57’ E). The nematode cultures were maintained on sloppy (1%) nutrient: bacto agar media (ratio of 1:4) (Moens and Vincx, 1998) with unidentified bacteria from their natural habitat under standardized conditions (temperature: 20°C; salinity: 25) for many generations before the start of the experiments.
2.2 Food sources for nematodes
The bacterial food sources for nematodes used in the present experiments were selected and prepared as described in Guden et al. (2021). Briefly, we prepared monospecific liquid cultures in sterile marine broth (Difco) of twenty-five marine bacterial strains (Supplementary Material, Supplementary Table 1). These bacteria were among the most abundant bacterial taxa found in the microbiome sensu lato of specimens of at least one cryptic species of L. marina in the field (Derycke et al., 2016). Bacteria were harvested from the liquid cultures by centrifugation (2,000 rpm; 20-min) after 96 h of shake-incubation (180 rpm) at 37°C. The growth medium (supernatant) was removed, whereafter the bacteria (pellet) were washed twice in sterile Artificial Seawater (ASW; salinity of 25) (Dietrich and Kalle, 1957). Finally, the suspensions of each bacterial strain were resuspended in ASW, and cell density was measured using a Bio UV visible spectrophotometer at OD600 (optical density at 600 nm). The cell density for each bacterial strain was diluted to ca. 3 × 109 cells ml-1, which is known to support good fitness and active population growth of L. marina (dos Santos et al., 2008; De Meester et al., 2011; Guden et al., 2021).
Three food-diversity treatments were prepared by mixing culture aliquots of 5, 10 and 15 bacterial strains for ‘low diversity’, ‘medium diversity’, and ‘high diversity’ food treatments, respectively (Figure 1B). Each bacterial mixture was prepared in such a way that approximately equal cell numbers of all bacterial strains were present, and added up to a final density of ca. 3 × 109 cells ml-1. Since we were interested in the effect of food diversity, we focused on the number of bacterial strains regardless of their identity. For this, bacteria were assigned to replicates of each food-diversity treatment by a separate random draw of the appropriate number of strains from our pool of twenty-five bacterial strains. We used frozen-and-thawed Escherichia coli (strain K12, density of ca. 3 × 109 cells ml-1) as a control food treatment, which has been used as a suitable food source in various experiments with L. marina (Moens and Vincx, 1998; dos Santos et al., 2008; De Meester et al., 2011).
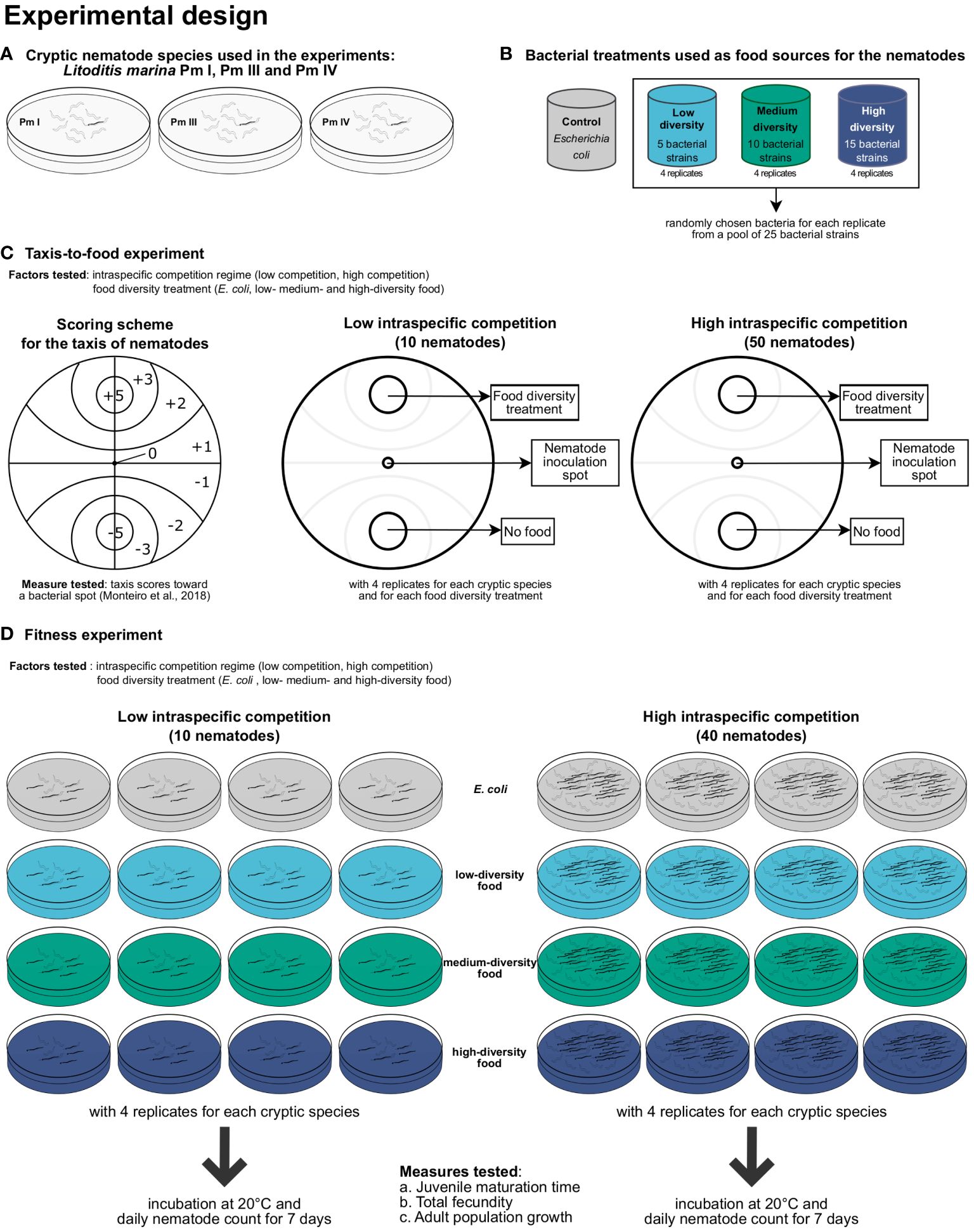
Figure 1 Summary of the experimental design for testing the effects of intraspecific competition and food diversity on L. marina. The (A) three cryptic species of L. marina and the (B) four food (bacteria)-diversity treatments used in the experiments are shown, including the setups for the (C) taxis-to-food and (D) fitness experiments.
2.3 Experimental design
A summary of the experimental design can be found in Figure 1.
2.3.1 Taxis-to-food experiments
The effects of intraspecific competition and food diversity on taxis to food of the different cryptic species of L. marina were investigated according to the protocol described in detail by Guden et al. (2021). In short, Petri dishes of 9 cm internal diameter were bottom-covered with 18 ml of 1% bacto-agar medium (salinity of 25; pH of 7.5–8). Two excavations with a diameter of 1.5 cm were created on opposite sides of the plate at equal distance from the center (Figure 1C). One excavation (food spot) was filled with 150 µl of bacterial suspensions (E. coli, ‘low diversity’, ‘medium diversity’, or ‘high diversity’), whereas the opposite excavation (control spot) did not contain any food and was only filled with 150 µl of sterile ASW. The food treatment with frozen-and-thawed Escherichia coli (strain K12) in the food spot was used as a positive control, and a treatment without any bacterial spots but with two spots of ASW was added as a negative control.
The low and high-intraspecific competition regimes for the taxis-to-food assays consisted of 10 and 50 adult nematodes of each cryptic species, respectively, which were manually picked and transferred to the center of the plate. The ‘low nematode density’ served as the setup with low intraspecific competition between nematodes of a single species, whereas the ‘high nematode density’ was the setup with high intraspecific competition. These intraspecific competition regimes were based on a previous experiment, which revealed differences in offspring performance across multiple generations of L. marina between these two nematode densities (Guden et al., in prep.). All the taxis plates were incubated in the dark at room temperature. After 5 h, the total numbers of nematodes per section on the plate were counted and multiplied by the corresponding section score based on the scoring scheme of Monteiro et al. (2018). The sum of these values was divided by the total number of inoculated nematodes to obtain one score for each plate. The attraction scores ranged from -5.0 (complete repulsion: all nematodes are in the control spot (= ASW)) to +5.0 (complete attraction: all nematodes are in the bacterial spot). The higher the score, the more the nematodes were attracted to the food spot.
2.3.2 Population fitness experiments
Petri dishes (9 cm i.d.) filled with 15 ml of 1% bacto-agar medium prepared with ASW (salinity of 25; pH of 7.5–8) were used as experimental microcosms (Figure 1D). Two different competition regimes (‘low nematode density’ and ‘high nematode density’) were established, with presumed varying strength of nematode intraspecific competition based on De Meester et al. (2015a). In the ‘low nematode density’, 5 adult males and 5 young reproductive females of a single cryptic species were transferred to the microcosms, and 200-µl aliquots of bacterial suspensions of each food treatment (density of ca. 3 × 109 cells ml-1) were spread over the agar surface. Nematodes were hand-picked from the stock cultures, washed in an embryo dish containing ASW with a salinity of 25, and transferred randomly on the agar plates. The setup with ‘high nematode density’ consisted of 40 nematodes (20 males and 20 females) of each cryptic species, which was also added with 200-µl suspensions of the food-diversity treatments. Each food-diversity treatment (E. coli, ‘low diversity’, ‘medium diversity’, and ‘high diversity’) was replicated four times for each cryptic species (Pm I, Pm III, and Pm IV) and competition regime (‘low nematode density’ and ‘high nematode density’).
Minimum juvenile development time, total fecundity, and adult population growth (total number of adults over time), which all contribute to population fitness (Moens and Vincx, 2000a), were quantified daily for a period of 7 days, corresponding to at least one and at most two generations in all species and food-diversity treatments (De Meester et al., 2015b). Minimum juvenile development time was defined as the time from the occurrence of the first juveniles until the appearance of the first F1-adult(s), which was used instead of minimum development time to take into account the differences in reproductive strategies between cryptic species (De Meester et al., 2012). In addition, the sum of the numbers of eggs and juveniles produced from the start of the incubation to the maturation of the first F1-offspring to adults was divided by the number of inoculated adult females (5 for ‘low nematode density’, 20 for ‘high nematode density’) to obtain an estimate of total fecundity per female (Moens and Vincx, 2000a). Note here that this is a conservative indication of total fecundity given that females might have already deposited eggs or juveniles prior to the start of the experimental incubation, and a (very) limited egg production may still occur after the first F1-offspring reach adulthood.
2.4 Data analyses
The effects of intraspecific competition and food diversity on taxis to food, minimum juvenile development time, total fecundity and population growth of L. marina were analyzed separately for each cryptic species. Parametric tests (ANOVA) were used after checking that the assumptions for normality (Shapiro-Wilk test) and/or homogeneity of variances (Levene’s test) were fulfilled; the data were transformed where necessary to fit the requirements for parametric tests. Pairwise post-hoc tests for significant terms (at α = 0.05) were conducted using the Tukey Honestly Significant Difference (HSD) test. Generalized Estimating Equation Models (GEEGLM) were conducted to test the effects of intraspecific competition and food diversity on adult population growth over time. All statistical analyses were conducted using R (R Core Team, 2023.
2.4.1 Taxis-to-Food Experiments
The effects of intraspecific competition and food diversity on taxis to food of each cryptic species were tested by comparing the attraction scores of the nematodes using a two-way ANOVA test with two fixed factors: competition regime (‘low nematode density’ and ‘high nematode density’) and food diversity (5 levels: ‘control (no food)’, ‘E. coli’, ‘low diversity’, ‘medium diversity’ and ‘high diversity’). Part of the taxis-to-food data (i.e., ‘high nematode density’) were published in Guden et al. (2021).
2.4.2 Population fitness experiments
2.4.2.1 Minimum juvenile development time
A two-way ANOVA test was conducted on the minimum juvenile development time as the dependent variable and with two fixed factors: competition regime (‘low nematode density’ and ‘high nematode density’) and food diversity (4 levels: ‘E. coli’, ‘low diversity’, ‘medium diversity’ and ‘high diversity’) for each cryptic species.
2.4.2.2 Total fecundity
A two-way ANOVA test was conducted on the data of total fecundity of each cryptic species, which was log(x + 1)-transformed for Pm III to conform to the assumptions of parametric tests. Competition regime (‘low nematode density’ and ‘high nematode density’) and food diversity (4 levels: E. coli, ‘low diversity’, ‘medium diversity’ and ‘high diversity’) were used as fixed factors.
2.4.2.3 Adult population growth
The effect of intraspecific competition and food diversity on the abundances of nematodes over time was analyzed separately for each cryptic species using Generalized Estimating Equation Models (GEEGLM) (Zuur et al., 2009) with the R package geepack v.1.3.2 (Yan, 2002; Yan and Fine, 2004; Halekoh et al., 2006), considering the dependence of counts at different time points. Two models were built separately on nematode counts for each cryptic species as dependent variables. In each model, the number of adult nematodes was entered as the dependent variable, competition regime (‘low nematode density’ and ‘high nematode density’) and food diversity (4 levels: E. coli, ‘low diversity’, ‘medium diversity’ and ‘high diversity’) as fixed factors, and time as a co-variable. A log-transformation [log(x + 1)] was performed on the count data of adult nematodes for Pm I and Pm IV to normalize the data; a quadratic model was used for Pm I and Pm III, whereas a linear model was used for Pm IV. Replicate sample IDs were also added to the model in the ‘id’ argument to incorporate time-repeated measurements of the same population. Autoregressive correlation structure (ar1) was specified in the model to account for co-variance of the counts from different time points. The models were validated by checking the residuals against the fitted values to be randomly scattered, and by performing a Shapiro-Wilk test on residuals. The Quasilikelihood under the Independence model Criterion (QIC) was used to select the most appropriate model for each cryptic species; the model with the smallest QIC measure was finally preferred. Significance of the results is given by the Wald Chi-Square test statistic for the explanatory variables at α = 0.05. A post-hoc Tukey HSD test was performed for pairwise comparisons of the significant terms using the R package emmeans (Lenth, 2021).
3 Results
3.1 Effects of intraspecific competition and food diversity on taxis to food
Significant interaction effects of intraspecific competition and food diversity were found in taxis to food of Pm III and Pm IV, whereas neither the individual factors nor its interaction had significant effects on Pm I (Table 1; Figure 2). Generally, we found higher taxis of L. marina toward all bacterial spots (E. coli, low-, medium- and high-diversity food) compared to the negative control (no food) in both competition regimes. In both Pm III and Pm IV, taxis to food did not significantly differ between food-diversity treatments under low intraspecific competition (all p < 0.05), but we found significantly different taxis depending on food diversity under high intraspecific competition. In the high-intraspecific competition regime, Pm III exhibited significantly higher taxis to medium and high-diversity food compared to E. coli (E vs. M: p = 6.2e-06; E vs. H: p = 0.007) and low-diversity food (L vs. M: p = 6.3e-07; L vs. H: p = 0.0006). On the other hand, Pm IV showed significantly higher taxis to medium-diversity food compared to E. coli, (p = 1.3e-05), low-diversity food (p = 1.5e-05) and high-diversity food (p = 1.4e-07), whereas taxis to food did not significantly differ between the other food-diversity treatments (all p > 0.05).
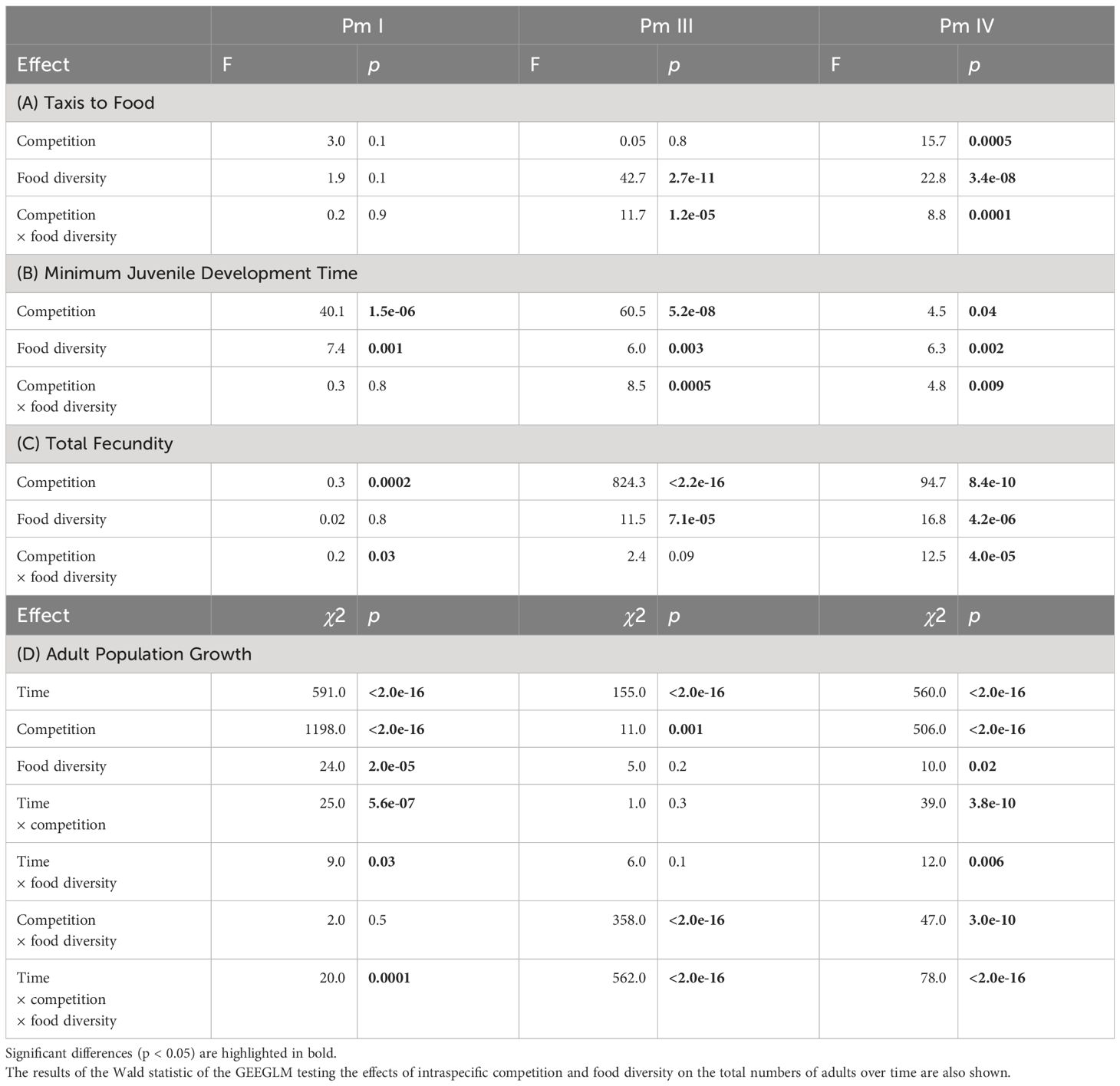
Table 1 Summary of the results of two-way ANOVA tests on the effects of intraspecific competition (low and high nematode density) and food diversity (E. coli, low-, medium-, and high-diversity food) on taxis to food, minimum juvenile development time and total fecundity for each cryptic species (Pm I, Pm III and Pm IV) of L. marina.
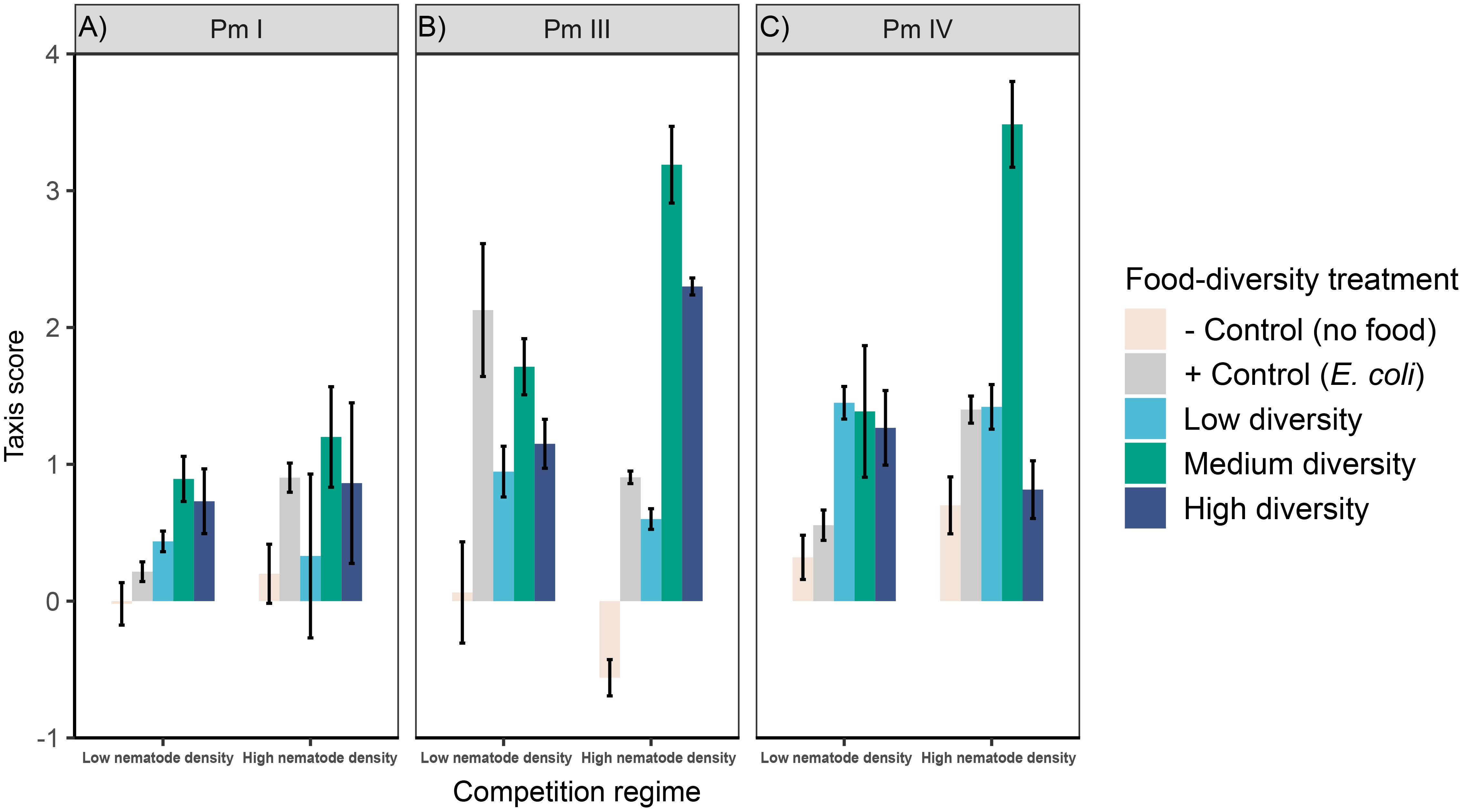
Figure 2 Taxis to food of L. marina in different intraspecific competition regimes and food-diversity treatments. Taxis-to-food scores (mean ± SE) for the three cryptic species of L. marina [(A) Pm I, (B) Pm III and (C) Pm IV] in different competition regimes (low nematode density and high nematode density) and food-diversity treatments (E. coli, low-diversity food, medium-diversity food, and high-diversity food) are shown (n = 4).
3.2 Effects of food diversity and intraspecific competition on life-history traits
3.2.1 Minimum juvenile development time
Intraspecific competition, food diversity and the interaction between the two factors had significant effects on the minimum juvenile development time of Pm III and Pm IV, whereas only the effects of individual factors were significant in Pm I (Table 1; Figure 3). Irrespective of food diversity, Pm I exhibited shorter minimum juvenile development time under high intraspecific competition than under low competition. In addition, Pm I showed significantly faster juvenile maturation time in high-diversity food compared to E. coli (p = 0.004) and low-diversity food (p = 0.004) irrespective of competition regimes, while no significant differences were observed between high and medium-diversity food (p = 0.6). For both Pm III and Pm IV, minimum juvenile development time did not significantly differ between food-diversity treatments under low intraspecific competition (all p > 0.05). In contrast, under high intraspecific competition, both Pm III and Pm IV exhibited faster juvenile maturation time in medium- and high-diversity food among all food-diversity treatments (all p < 0.05).
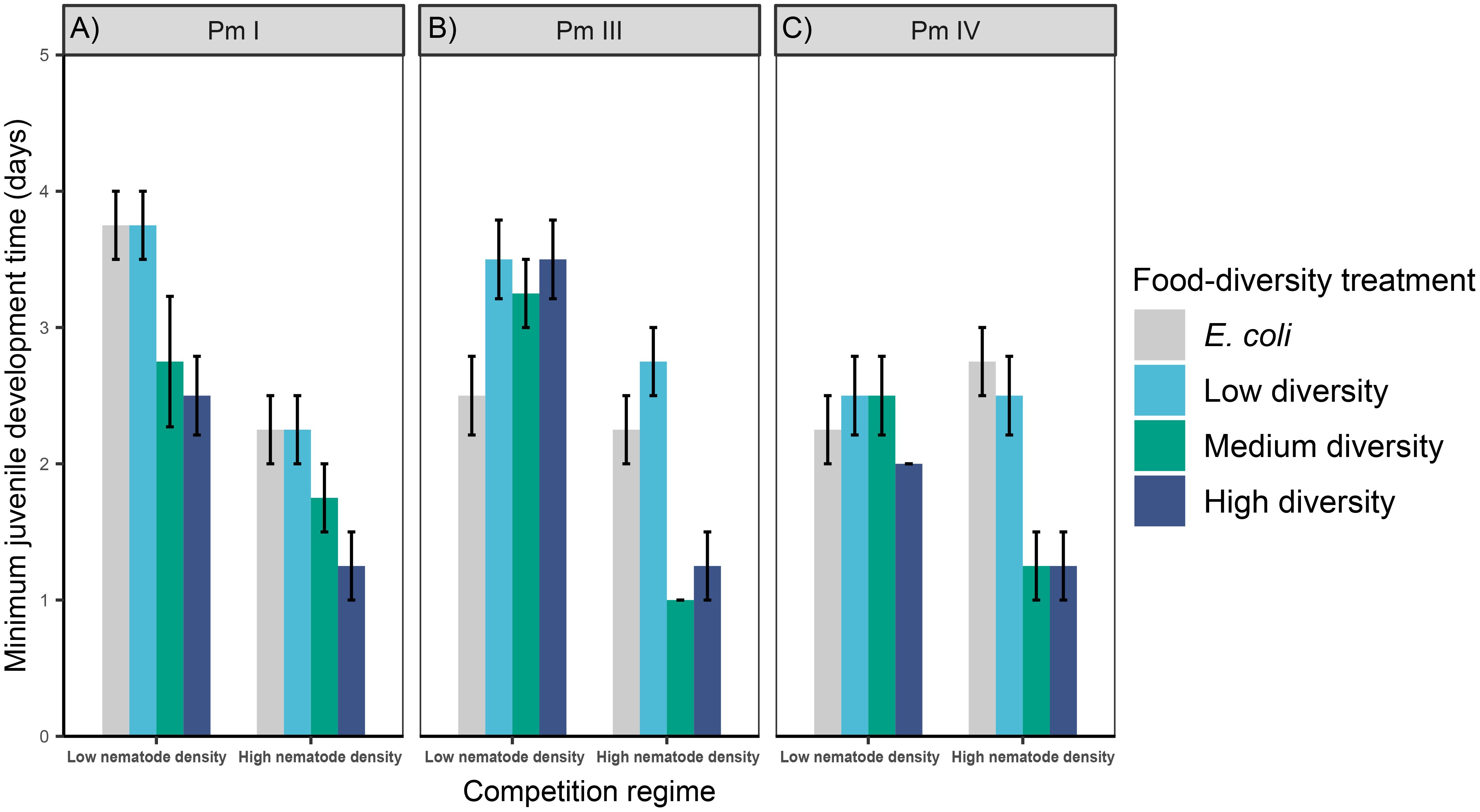
Figure 3 Minimum juvenile development time of L. marina in different intraspecific competition regimes and food-diversity treatments. The rates of minimum juvenile development time (mean ± SE) for the three cryptic species of L. marina [(A) Pm I, (B) Pm III and (C) Pm IV] in different competition regimes (low nematode density and high nematode density) and food-diversity treatments (E. coli, low-diversity food, medium-diversity food, and high-diversity food) are shown (n = 4).
3.2.2 Total fecundity
We found significant interaction effects of intraspecific competition and food diversity on the total fecundity of Pm I and Pm IV but not of Pm III; both individual factors had significant effects on Pm III as well as on Pm IV, whereas only intraspecific competition had a significant effect on Pm I (Table 1; Figure 4). In both competition regimes, no significant differences were found between food-diversity treatments in Pm I (all p < 0.05); the significant interaction effect of intraspecific competition and food diversity only revealed significantly lower fecundity under high intraspecific competition compared to low competition in the low-diversity food treatment (p = 0.02) and E. coli (p = 0.02). In Pm III, we observed significantly lower fecundity under high intraspecific competition than under low competition irrespective of food diversity. Moreover, Pm III exhibited significantly higher fecundity in high- and low-diversity food compared to E. coli (E vs. H: p = 0.0002; E vs. L: p = 0.001) and medium-diversity food (M vs. H: p = 0.005; M vs. L: p = 0.03) regardless of competition regimes. On the other hand, Pm IV exhibited significantly higher fecundity both in medium and low-diversity food compared to E. coli (E vs. M: p = 0.0006; E vs. L: p = 7.0e-07) and high-diversity food (H vs. M: p = 0.007; H vs. L: p = 6.6e-06) but only under low intraspecific competition; no significant differences between food-diversity treatments were observed under high intraspecific competition (all p > 0.05).
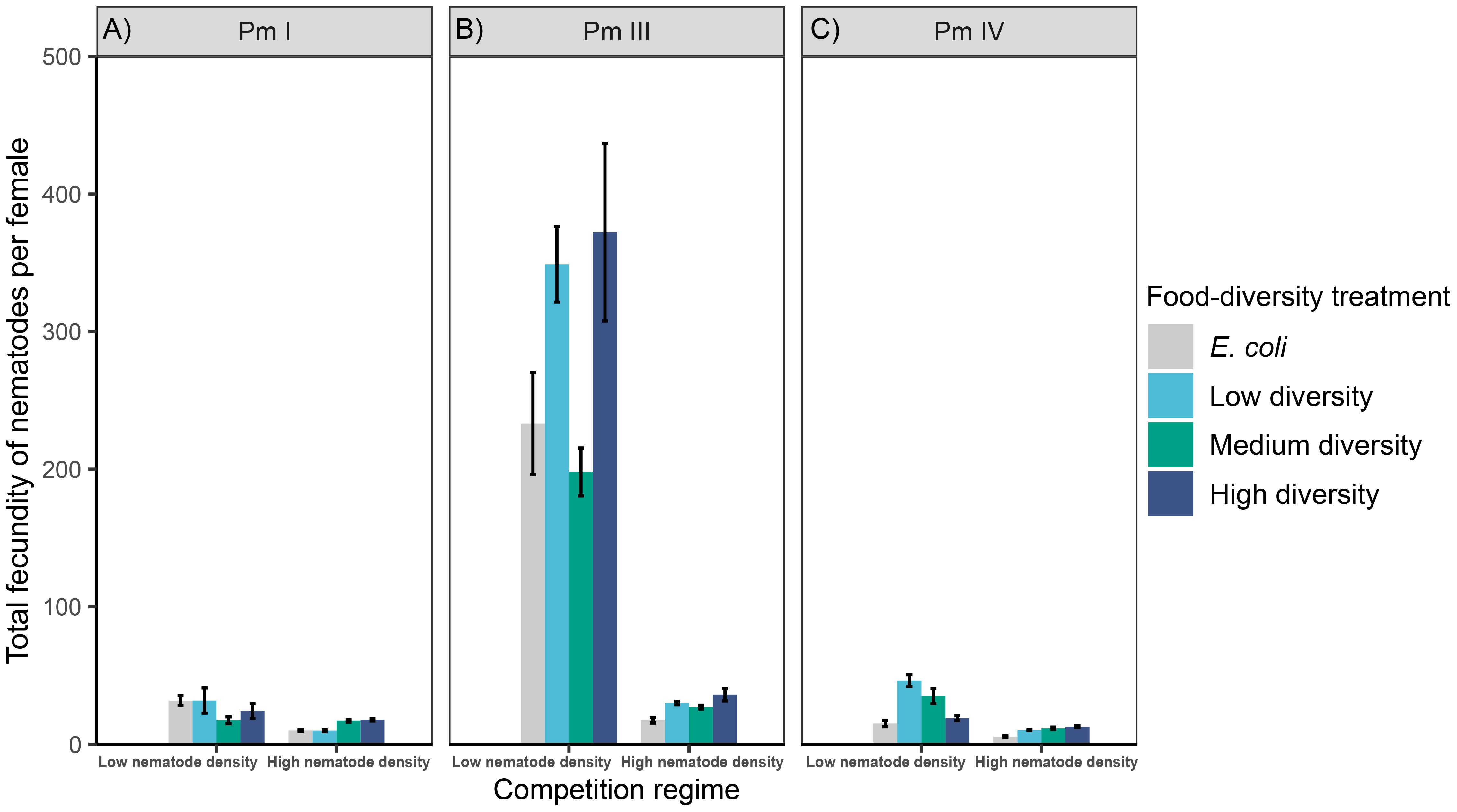
Figure 4 Total fecundity of L. marina in different intraspecific competition regimes and food-diversity treatments. The total number of offspring (eggs + juveniles) per female produced over the interval from the start of the incubation to the maturation of the first F1-offspring to adults (mean ± SE) for the three cryptic species of L. marina [(A) Pm I, (B) Pm III and (C) Pm IV] in different competition regimes (low nematode density and high nematode density) and food-diversity treatments (E. coli, low-diversity food, medium-diversity food, and high-diversity food) are shown (n = 4).
3.3 Effects of intraspecific competition and food diversity on adult population growth
The adult population abundances of each cryptic species of L. marina were significantly influenced by the interaction of time, intraspecific competition and food diversity (Table 1; Figure 5). In the low-intraspecific competition regime, Pm I yielded significantly higher adult population growth in high-diversity food compared to E. coli (p = 0.04), but they performed equally well in low-, medium- and high-diversity food (all p > 0.05). In contrast, in the high-intraspecific competition regime, Pm I had the highest adult population growth in high-diversity food among all food-diversity treatments (H vs. E: p = 0.0001; H vs. L: p = 0.0001; H vs. M: p = 0.0001), and the lowest adult population growth in E. coli (E vs. L: p = 0.01; E vs. M: p = 0.01; E vs. H: p = 0.0001). In Pm III, adult population growth was highest in E. coli and lowest in medium-diversity food among all food-diversity treatments under low intraspecific competition (all p < 0.05), while the highest adult population growth was achieved in the two most diverse food sources under high intraspecific competition (all p < 0.05). In Pm IV, adult population growth was highest in low-diversity food under low intraspecific competition, whereas this species performed best in high-diversity food under high intraspecific competition (all p < 0.05).
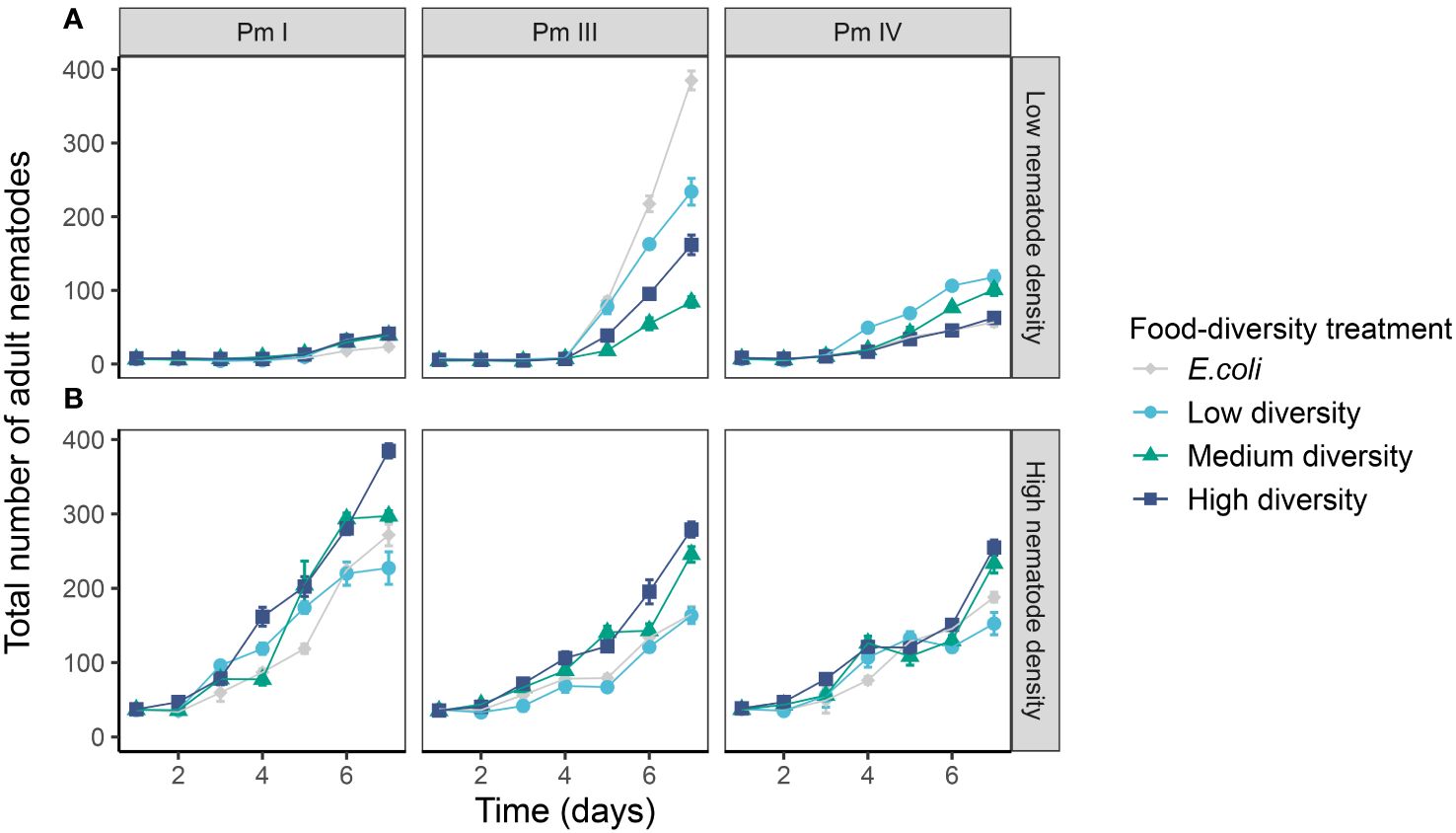
Figure 5 Adult population growth of L. marina in different intraspecific competition regimes and food-diversity treatments. The total number of adult nematodes over time (mean ± SE) for the three cryptic species of L. marina (Pm I, Pm III and Pm IV) in different competition regimes [(A) low nematode density and (B) high nematode density] and food-diversity treatments (E. coli, low-diversity food, medium-diversity food, and high-diversity food) are shown (n = 4).
4 Discussion
Intraspecific competition is considered a major driver of trophic niche differentiation (Araújo et al., 2011), but its impact on population dynamics may depend on its interaction with resource diversity (Costa-Pereira et al., 2017). Here, we demonstrate that food diversity can mitigate the effects of intraspecific competition on foraging behavior and fitness of the cryptic species of the nematode morphospecies complex L. marina, but this response can also differ considerably between these morphologically similar nematode species. A summary of the results for each cryptic species can be found in Table 2.
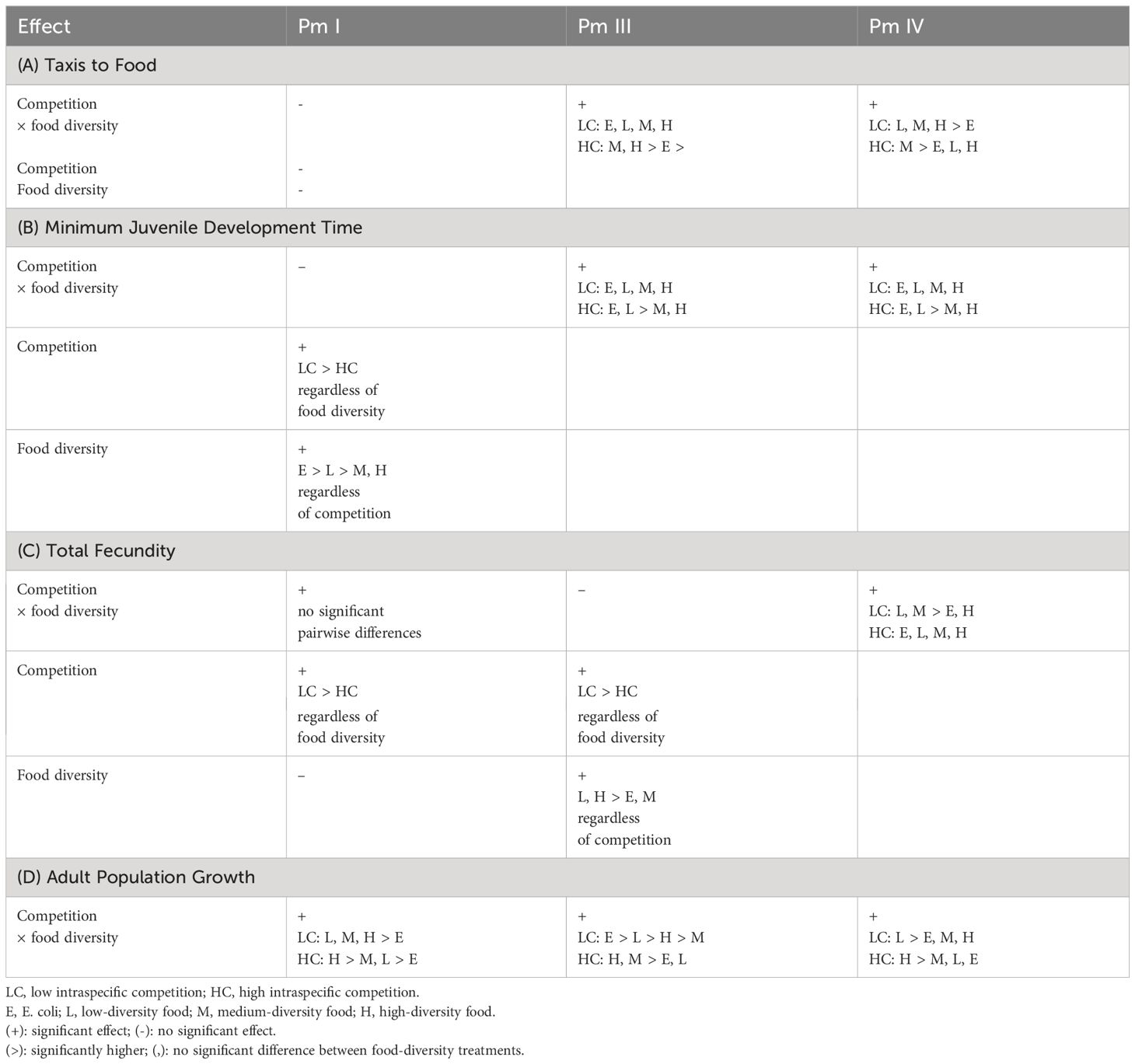
Table 2 Summary of the results showing the effects of intraspecific competition and food diversity on the foraging behavior (taxis to food) and fitness (minimum juvenile development time, total fecundity and adult population growth) of each cryptic species (Pm I, Pm III and Pm IV) of L. marina.
4.1 Intraspecific competition and food diversity can alter the foraging behavior of L. marina
Theoretical and empirical studies support the notion that intraspecific competition promotes population niche expansion since the increase in resource competition will drive individuals to feed on alternative resources (Van Valen, 1965; Svanbäck and Bolnick, 2007; Tinker et al., 2008; Svanbäck et al., 2011). Food diversity (often termed ‘ecological diversity’) can also expand the trophic niche of a population because higher food diversity creates greater foraging possibilities, enhancing individual diet specialization (Evangelista et al., 2014; Newsome et al., 2015; Costa-Pereira et al., 2017; Bolnick and Ballare, 2020). Animals have been reported to behaviorally alter their diet in response to both intraspecific competition (Svanbäck and Bolnick, 2007; Tinker et al., 2008; Lamb et al., 2017) and food diversity (Parent et al., 2014).
Consistent with these findings, we found that L. marina is capable of adopting their behavioral response towards food spots depending on intraspecific competition and food diversity (Figure 2). When subject to high intraspecific competition, Pm III preferred medium- and high-diversity food over low-diversity food, but they did not exhibit such preferences under low intraspecific competition. This demonstrates that high intraspecific competition drives stronger selection for a more diverse food source in Pm III; such diet plasticity could be an important foraging strategy to minimize the effects of intraspecific competition via population niche expansion (Svanbäck and Bolnick, 2007; Toscano et al., 2016). A population’s trophic niche can expand either through an increase in individual diet width when each individual adds new types of food to its diet, or through an increase in among-individual diet width wherein each individual feeds on a subset of the resource that is different from other subsets eaten by other individuals (Araújo et al., 2011). A large body of evidence supports a positive relationship between population niche expansion and among-individual diet variation (Svanbäck and Bolnick, 2007; Araújo et al., 2009), which promotes individual diet specialization (Araújo et al., 2011) that results in niche diversification (Bolnick et al., 2003; Svanbäck and Bolnick, 2005; Bolnick et al., 2007). Although our study does not allow us to infer the mechanism for population niche expansion in Pm III, microbiome analyses provide evidence that such expansion arises through among-individual diet variation (De Meester et al., in prep.). As part of future work, we suggest to complement these results with stable isotope analysis to confirm individual diet specialization in L. marina. The carbon and nitrogen isotopic ratios are standard methods used to elucidate patterns in food webs (Bearhop et al., 2004), such as intrapopulation variation in feeding strategies (Estes et al., 2003; Gerardo Herrera et al., 2008). Integrating different approaches may enhance our understanding of population trophic niche expansion, particularly on the importance of the dimensionality of individual niche variation (Ingram et al., 2018).
Nonetheless, the effects of intraspecific competition and food diversity on the foraging strategies of L. marina can also vary considerably between cryptic species. While Pm III exhibited food preference only under high intraspecific competition, Pm IV preferred a medium or highly diverse food source even under low intraspecific competition. Diverse food sources may offer a more complete range of nutritional resources (‘balanced-diet hypothesis’) (DeMott, 1998; Duffy, 2002). While it would have been more informative if we were able to assess the nutritional quality of each bacterial strain used in the experiments, our preliminary analyses showed that the individual bacterial strains can support nematode growth. Interestingly, when subjected to high intraspecific competition, Pm IV preferred medium-diversity food over both low and high-diversity food spots. Nematodes rely on their neurosensory abilities in their food-search activities (Gaugler and Bilgrami, 2004); such abilities may work best when there is sufficient diversity of stimuli, but less optimal when a large number of different stimuli must be “processed” (Guden et al., 2021).
In sharp contrast to Pm III and Pm IV, neither intraspecific competition nor food diversity influenced the food preference of Pm I. The latter consumes a wide diversity of bacterial strains, and is probably a more generalist feeder compared to Pm III (Derycke et al., 2016). This may explain why it did not exhibit food preferences regardless of competition regimes, and suggests that food diversity may be less biologically important for generalist species as also observed in the tetra fish Astyanax lacustris (Costa-Pereira et al., 2017).
4.2 Food diversity can mitigate the effects of intraspecific competition on population fitness of L. marina
Resource competition dramatically impacts multiple aspects of population fitness (Parent et al., 2014; Evangelista et al., 2020). Here, we show that intraspecific competition has negative effects on different life-history characteristics of L. marina, but these fitness effects can be mitigated by food diversity. Under high intraspecific competition, we observed enhanced population growth of all cryptic species at a more diverse food source (Figure 5). These results are striking because the different replicates of each diversity treatment had different combinations of bacterial strains, which highlight the importance of diversity effect over strain effect. Specifically, we observed that Pm I and Pm IV had the highest adult population growth in high-diversity food among all food-diversity treatments under high intraspecific competition, while Pm III performed equally well in high- and medium-diversity food. The high adult population abundance of Pm III and Pm IV in the high-diversity food treatment corresponded with the fast juvenile maturation (Figure 3) of both cryptic species in this food source under high intraspecific competition. These results support population niche expansion through increased individual specialization as a response to alleviate intraspecific competition in L. marina (De Meester et al., in prep.), which can provide more scope for intraspecific complementarity (Araújo et al., 2011; Hausch et al., 2018). Furthermore, the fitness performance of Pm III and Pm IV in a more diverse food source also coincided with the food preferences of both cryptic species in the presence of high intraspecific competition (Figure 2). This is in accordance with a wide variety of empirical evidence supporting the preference-performance hypothesis, which demonstrates that animals tend to feed optimally by choosing resources that maximize offspring performance (Gripenberg et al., 2010).
The beneficial effect of a more diverse food source on adult population abundances of L. marina did not result from a higher fecundity on a more diverse diet, but rather from a faster development. Indeed, Pm I and Pm III exhibited lower fecundity under high intraspecific competition than under low competition regardless of food diversity, while the fecundity of Pm IV did not differ between food-diversity treatments in the high-intraspecific competition regime (Figure 4). Both Pm III and Pm IV may invest less energy in reproduction with increasing intraspecific competition, and instead maximize their energy intake by finding their preferred food and by increasing population growth through faster juvenile maturation, as predicted by optimal foraging theory (Stephens and Krebs, 1986; Sih and Christensen, 2001). Reproduction is costly, both in terms of energy (Stevenson and Bryant, 2000) and fitness (Visser and Lessells, 2001). In Pm I, intraspecific competition had pronounced negative effects both on juvenile maturation (Figure 3) and fecundity (Figure 4) regardless of food-diversity treatments. In addition, Pm I did not exhibit differences in taxis to food between food-diversity treatments (Figure 2); such lack of preference may indicate nonoptimal feeding (Parent et al., 2014) that might have impacted the ability of Pm I to cope with intraspecific competition. In order to escape intraspecific competition, Pm I may instead invest their energy on dispersal (which can be triggered by intraspecific competition, De Meester et al., 2015a), particularly in quickly dispersing to patches with a more diverse food (Guden et al., in prep.).
4.3 Do neutral or niche-based mechanisms underlie the coexistence of cryptic species?
The present study confirms that there is strong intraspecific competition in L. marina, which affects foraging behavior and population dynamics of the cryptic species. We also demonstrate that the effects of food diversity on the foraging behavior and fitness of the cryptic species can alter the outcome of intraspecific competition. The differential response of L. marina in relation to intraspecific competition can be a mechanism of niche differentiation between the cryptic species, which may allow them to coexist in the field. Our previous study showing that food diversity also has a significant effect on the interspecific interactions of L. marina further supports the crucial role of food diversity in community dynamics and coexistence of L. marina in the field (Guden et al., 2021). L. marina lives on both living and decaying macro-algae in the littoral zone, a highly heterogeneous environment with abiotic and biotic conditions that fluctuate at different spatial and temporal scales (Moens and Vincx, 2000a, b). The microbial biofilms attached on the surfaces of macro-algae are the most important food sources for L. marina, but these bacterial communities differ between algal species and vary temporally (Lachnit et al., 2011). In addition, bacterial communities may vary between two nearby algal patches, and even between different algal structures. For instance, we found distinct microhabitat preferences between the cryptic species of L. marina, which may be associated with the differences in bacterial communities between microhabitats (Guden et al., 2018). Furthermore, the ‘lifespan’ of macro-algae may also likely affect the composition of bacterial communities: when an algal plant dies or when decomposing algae deteriorates, temporal variability in bacterial community composition is formed, which in turn, may change the availability and/or quality of resources for nematodes (De Meester, 2016). Hence, in the ephemeral habitat where L. marina lives, with rapid changes in abiotic regimes and biotic interactions (Moens and Vincx, 2000a, b), species coexistence may thus be mediated among other things by resource diversity.
Our findings are all the more relevant because the strong intraspecific competition in L. marina suggests that neutral dynamics may also play a role in the co-occurrence of the different cryptic species in the field. Neutral theory postulates ecological equivalence among competing species, and proposes that species can coexist for extended periods of time as their relative abundances change through a completely stochastic drift process (Bell, 2001; Hubbell, 2006). Coexistence will therefore be dependent on neutral dynamics if species differences are minimal and/or if intraspecific competition is as high as, or higher than interspecific competition (Chesson, 2000; McPeek, 2005; Leibold and McPeek, 2006). In this respect, intraspecific variation is expected to break down competitive hierarchies, making competitive exclusion less likely or less rapid (Hubbell, 2006; Fridley et al., 2007). While the different cryptic species of L. marina exhibit ecological- and functional-trait differences (De Meester et al., 2011, De Meester et al., 2012; De Meester et al., 2015a, b, De Meester et al., 2016; Guden et al., 2018, Guden et al., 2021), which provide support for niche differentiation as a driver of species coexistence, a competitive intransitive network (i.e., non-hierarchical competition) likely exists in L. marina (De Meester, 2016). Such competitive intransitivity indicates that there is no single best competitor, which can slow down the process of competitive exclusion and make neutral processes more important (Laird and Schamp, 2006, 2008; Soliveres and Allan, 2018). To confirm this, investigating the relative strength of intra- and interspecific competition between the cryptic species of L. marina is necessary for future studies. If intraspecific competition is as high as, or higher than interspecific competition, then coexistence will also be dependent on neutral dynamics (Chesson, 2000; McPeek, 2005; Leibold and McPeek, 2006).
While the current study has broadened our understanding on diversity maintenance in ecological communities, we do acknowledge certain methodological limitations of our work. First, our findings are based on experiments conducted in laboratory conditions using microcosms with a limited number of replicates. Complex and heterogeneous natural ecosystems can be simplified and simulated using microcosms (Cao et al., 2021), but insights using this approach may not entirely reflect larger scale processes in the real world (Benton et al., 2007). Therefore, caution is due when extrapolating our findings to field conditions. Nevertheless, while microcosms do not mirror nature, they can deepen our understanding of natural processes by which we can develop and test ecological theories and gain knowledge on how species coexist in the field (Fraser, 1999; Benton et al., 2007). A second limitation is the level of resource diversity used in our experimental work. In nature, the different cryptic species of L. marina are definitely exposed to a much higher diversity of resources than what was used in our study, and as such they may respond differently. Although we can never fully mimic the diversity of resources in the real world, our study reveals that resource diversity plays an important role on the behavior and interactions of different cryptic species, and thus may mediate their coexistence in the field.
5 Conclusion
Our study provides empirical evidence that food diversity can alleviate intraspecific competition in L. marina through its effects on food preference and fitness performance, but the response of nematodes can also vary considerably between cryptic species. L. marina is capable of adopting their food preference depending on intraspecific competition and food diversity. High intraspecific competition drives stronger selection for a more diverse food source in Pm III and Pm IV, while Pm I lacks food preference regardless of competition regimes and food diversity. Despite the species-specific differences in food preference, all cryptic species exhibit enhanced adult population growth on a more diverse food source under high intraspecific competition, which may reflect population niche expansion through individual diet specialization. The effects of food diversity on foraging behavior and fitness of L. marina, which alters the outcome of intraspecific competition, suggests that food diversity plays an important role in species distribution, population dynamics, and diversity maintenance in ecological communities.
Data availability statement
The datasets presented in this study can be found in online repositories. All data of the study is publicly available in the Integrated Marine Information System (IMIS) database (VLIZ): https://doi.org/10.14284/611.
Ethics statement
The manuscript presents research on animals that do not require ethical approval for their study.
Author contributions
RG was heavily involved in all aspects of the present work, conducted the laboratory experiments, performed the data analyses, and wrote the original draft. RG and TM conceived this study and its sampling design. TM provided funding for this research. SD and TM contributed to the writing of the manuscript. All authors contributed to the article and approved the submitted version.
Funding
The author(s) declare financial support was received for the research, authorship, and/or publication of this article. RG benefitted from a Ph.D. Scholarship from Ghent University (BOF) during the period of this study. The results of this study were obtained using infrastructure of EMBRC Belgium, funded by the Flemish Science Fund FWO (project G0H3817N). Specific funding for the laboratory experiments was provided by the FWO through project 3G.0192.09, and by the BiodivERsA project BIO-Tide ‘The role of microbial biodiversity in the functioning of marine tidal flat sediments’ (EU Horizon 2020 ERA-Net COFUND), with financial support of FWO (ERA Biodiversa 3G0H6816) and the Belgian Federal Science Policy Belspo (BRAIN-be contract BR/175/A1/BIO-Tide-BE). Additional funding was provided by Ghent University through GOA-project (17GOA-026) of the university’s Special Research Fund.
Acknowledgments
Nele De Meester is gratefully acknowledged for her helpful feedback on the experimental design.
Conflict of interest
The authors declare that the research was conducted in the absence of any commercial or financial relationships that could be construed as a potential conflict of interest.
Publisher’s note
All claims expressed in this article are solely those of the authors and do not necessarily represent those of their affiliated organizations, or those of the publisher, the editors and the reviewers. Any product that may be evaluated in this article, or claim that may be made by its manufacturer, is not guaranteed or endorsed by the publisher.
Supplementary material
The Supplementary Material for this article can be found online at: https://www.frontiersin.org/articles/10.3389/fmars.2024.1240802/full#supplementary-material
References
Adey A. K., Larson E. R. (2021). Testing the relationship between intraspecific competition and individual specialization across both behavior and diet. Ecol. Evol. 11, 11310–11322. doi: 10.1002/ece3.7916
Andow D. A. (1991). Vegetational diversity and arthropod population response. Annu. Rev. Entomology 36, 561–586. doi: 10.1146/annurev.en.36.010191.003021
Araújo M. S., Bolnick D. I., Layman C. A. (2011). The ecological causes of individual specialisation. Ecol. Lett. 14, 948–958. doi: 10.1111/j.1461-0248.2011.01662.x
Araújo M. S., Bolnick D. I., Martinelli L. A., Giaretta A. A., Dos Reis S. F. (2009). Individual-level diet variation in four species of Brazilian frogs. J. Anim. Ecol. 78, 848–856. doi: 10.1111/j.1365-2656.2009.01546.x
Bearhop S., Adams C. E., Waldron S., Fuller R. A., Macleod H. (2004). Determining trophic niche width: A novel approach using stable isotope analysis. J. Anim. Ecol. 73, 1007–1012. doi: 10.1111/j.0021-8790.2004.00861.x
Benton T. G., Solan M., Travis J. M., Sait S. M. (2007). Microcosm experiments can inform global ecological problems. Trends Ecol. Evol. 22, 516–521. doi: 10.1016/j.tree.2007.08.003
Birnie-Gauvin K., Peiman K. S., Raubenheimer D., Cooke S. J. (2017). Nutritional physiology and ecology of wildlife in a changing world. Conserv. Physiol. 5, 1–18. doi: 10.1093/conphys/cox030
Bolnick D. I., Ballare K. M. (2020). Resource diversity promotes among-individual diet variation, but not genomic diversity, in lake stickleback. Ecol. Lett. 23, 495–505. doi: 10.1111/ele.13448
Bolnick D. I., Svanbäck R., Araújo M. S., Persson L. (2007). Comparative support for the niche variation hypothesis that more generalized populations also are more heterogeneous. Proc. Natl. Acad. Sci. United States America 104, 10075–10079. doi: 10.1073/pnas.0703743104
Bolnick D. I., Svanbäck R., Fordyce J. A., Yang L. H., Davis J. M., Hulsey C. D., et al. (2003). The ecology of individuals: Incidence and implications of individual specialization. Am. Nat. 161, 1–28. doi: 10.1086/343878
Brook B. W., Bradshaw C. J. (2006). Strength of evidence for density dependence in abundance time series of 1198 species. Ecology 87, 1445–1451. doi: 10.1890/0012-9658(2006)87[1445:SOEFDD]2.0.CO;2
Cao Z., Li P., Li Z. H. (2021). A latest review on the application of microcosm model in environmental research. Environ. Sci. pollut. Res. 28, 60438–60447. doi: 10.1007/s11356-021-16424-7
Chesson P. (2000). General theory of competitive coexistence in spatially-varying environments. Theor. Population Biol. 58, 211–237. doi: 10.1006/tpbi.2000.1486
Costa-Pereira R., Tavares L. E., de Camargo P. B., Araújo M. S. (2017). Seasonal population and individual niche dynamics in a tetra fish in the Pantanal wetlands. Biotropica 49, 531–538. doi: 10.1111/btp.12434
De Meester N. (2016). Unravelling coexistence of cryptic Litoditis marina species. Doctoral dissertation, Ghent University.
De Meester N., Derycke S., Bonte D., Moens T. (2011). Salinity effects on the coexistence of cryptic species: A case study on marine nematodes. Mar. Biol. 158, 2717–2726. doi: 10.1007/s00227-011-1769-5
De Meester N., Derycke S., Moens T. (2012). Differences in time until dispersal between cryptic species of a marine nematode species complex. PloS One 7, 1–8. doi: 10.1371/journal.pone.0042674
De Meester N., Derycke S., Rigaux A., Moens T. (2015a). Active dispersal is differentially affected by inter- and intraspecific competition in closely related nematode species. Oikos 124, 561–570. doi: 10.1111/oik.01779
De Meester N., Derycke S., Rigaux A., Moens T. (2015b). Temperature and salinity induce differential responses in life histories of cryptic nematode species. J. Exp. Mar. Biol. Ecol. 472, 54–62. doi: 10.1016/j.jembe.2015.07.002
De Meester N., Gingold R., Rigaux A., Derycke S., Moens T. (2016). Cryptic diversity and ecosystem functioning: A complex tale of differential effects on decomposition. Oecologia 182, 559–571. doi: 10.1007/s00442-016-3677-3
DeMott W. R. (1998). Utilization of a cyanobacterium and a phosphorus-deficient green alga as complementary resources by daphnids. Ecology 79, 2463–2481. doi: 10.1890/0012-9658(1998)079[2463:UOACAA]2.0.CO;2
Derycke S., Backeljau T., Vlaeminck C., Vierstraete A., Vanfleteren J., Vincx M., et al. (2006). Seasonal dynamics of population genetic structure in cryptic taxa of the Pellioditis marina complex (Nematoda: Rhabditida). Genetica 128, 307–321. doi: 10.1007/s10709-006-6944-0
Derycke S., De Meester N., Rigaux A., Creer S., Bik H., Thomas W. K., et al. (2016). Coexisting cryptic species of the Litoditis marina complex (Nematoda) show differential resource use and have distinct microbiomes with high intraspecific variability. Mol. Ecol. 25, 2093–2110. doi: 10.1111/mec.13597
Derycke S., Remerie T., Backeljau T., Vierstraete A., Vanfleteren J., Vincx M., et al. (2008). Phylogeography of the Rhabditis (Pellioditis) marina species complex: Evidence for long-distance dispersal, and for range expansions and restricted gene flow in the Northeast Atlantic. Mol. Ecol. 17, 3306–3322. doi: 10.1111/j.1365-294X.2008.03846.x
Dietrich G., Kalle K. (1957). Allgemeine Meereskunde: Eine Einführung in die Ozeanographie. 8th edn (Berlin: Gebrüder Bornträger).
dos Santos G. A. P., Derycke S., Fonsêca-Genevois V. G., Coelho L. C. B. B., Correia M. T. S., Moens T. (2008). Differential effects of food availability on population growth and fitness of three species of estuarine, bacterial-feeding nematodes. J. Exp. Mar. Biol. Ecol. 355, 27–40. doi: 10.1016/j.jembe.2007.11.015
Duffy J. E. (2002). Biodiversity and ecosystem function: The consumer connection. Oikos 99, 201–219. doi: 10.1034/j.1600-0706.2002.990201.x
Estes J. A., Riedman M. L., Staedler M. M., Tinker M. T., Lyon B. E. (2003). Individual variation in prey selection by sea otters: Patterns, causes and implications. J. Anim. Ecol. 72, 144–155. doi: 10.1046/j.1365-2656.2003.00690.x
Evangelista C., Boiche A., Lecerf A., Cucherousset J. (2014). Ecological opportunities and intraspecific competition alter trophic niche specialization in an opportunistic stream predator. J. Anim. Ecol. 83, 1025–1034. doi: 10.1111/1365-2656.12208
Evangelista C., Vøllestad L. A., Pauli B. D., Edeline E. (2020). Density-dependent consequences of size-selective induced life-history changes to population fitness in Medaka (Oryzias latipes). Can. J. Fisheries Aquat. Sci. 77, 1741–1748. doi: 10.1139/cjfas-2019-0406
Fowler C. (1987). A review of density dependence in populations of large mammals (New York: Springer Science and Business Media).
Fraser L. H. (1999). The use of microcosms as an experimental approach to understanding terrestrial ecosystem functioning. Adv. Space Res. 24, 297–302. doi: 10.1016/S0273-1177(99)00317-8
Fridley J. D., Grime J. P., Bilton M. (2007). Genetic identity of interspecific neighbours mediates plant responses to competition and environmental variation in a species-rich grassland. J. Ecol. 95, 908–915. doi: 10.1111/j.1365-2745.2007.01256.x
Gaugler R., Bilgrami A. L. (2004). Nematode behaviour (Wallingford, UK: CABI publishing). doi: 10.1079/9780851998183.0000
Gerardo Herrera M., Korine C., Fleming T. H., Arad Z., Herrera M L. G., Korine C., et al. (2008). Dietary implications of intrapopulation variation in nitrogen isotope composition of an old world fruit bat. J. Mammalogy 89, 1184–1190. doi: 10.1644/07-MAMM-A-263.1
Gripenberg S., Mayhew P. J., Parnell M., Roslin T. (2010). A meta-analysis of preference-performance relationships in phytophagous insects. Ecol. Lett. 13, 383–393. doi: 10.1111/j.1461-0248.2009.01433.x
Guden R., Derycke S., Moens T. (2021). A multi-faceted approach to understand how resource diversity can mediate the coexistence of cryptic marine nematode species. Front. Mar. Sci. 8. doi: 10.3389/fmars.2021.777425
Guden R., Vafeiadou A., De Meester N., Derycke S., Moens T. (2018). Living apart-together: Microhabitat differentiation of cryptic nematode species in a saltmarsh habitat. PloS One 13, 1–18. doi: 10.1371/journal.pone.0204750
Halekoh U., Højsgaard S., Yan J. (2006). The R package geepack for Generalized Estimating Equations. J. Stat. Software 15, 1–11. doi: 10.18637/jss.v015.i02
Hart S. P., Schreiber S. J., Levine J. M. (2016). How variation between individuals affects species coexistence. Ecol. Lett. 19, 825–838. doi: 10.1111/ele.12618
Hausch S., Vamosi S. M., Fox J. W. (2018). Effects of intraspecific phenotypic variation on species coexistence. Ecology 99, 1453–1462. doi: 10.1002/ecy.2346
Hillebrand H., Shurin J. B. (2005). “Biodiversity and aquatic food webs,” in Aquatic Food Webs: An ecosystem approach. Eds. Belgrano A., Scharler U. M., Dunne J., Ulanowicz R. E. (Oxford University Press, Oxford), 184–198. doi: 10.1093/acprof:oso/9780198564836.003.0015
Hubbell S. P. (2006). Neutral theory and the evolution of ecological equivalence. Ecology 87, 1387–1398. doi: 10.1890/0012-9658(2006)87[1387:NTATEO]2.0.CO;2
Ingram T., Costa-Pereira R., Araújo M. S. (2018). The dimensionality of individual niche variation. Ecology 99, 536–549. doi: 10.1002/ecy.2129
Joshi J., Otway S. J., Koricheva J., Pfisterer A. B., Alphei J., Roy B. A., et al. (2004). “Bottom-up effects and feedbacks in simple and diverse experimental grassland communities,” in Insects and ecosystem function. Eds. Weisser W. W., Siemann E. (Springer- Verlag, Berlin, Heildelberg), 115–134. doi: 10.1007/978-3-540-74004-96
Lachnit T., Meske D., Wahl M., Harder T., Schmitz R. (2011). Epibacterial community patterns on marine macroalgae are host-specific but temporally variable. Environ. Microbiol. 13, 655–665. doi: 10.1111/j.1462-2920.2010.02371.x
Laird R. A., Schamp B. S. (2006). Competitive intransitivity promotes species coexistence. Am. Nat. 168, 182–193. doi: 10.1086/506259
Laird R. A., Schamp B. S. (2008). Does local competition increase the coexistence of species in intransitive networks? Ecology 89, 237–247. doi: 10.1890/07-0117.1
Lamb J. S., Satgé Y. G., Jodice P. G. (2017). Influence of density-dependent competition on foraging and migratory behavior of a subtropical colonial seabird. Ecol. Evol. 7, 6469–6481. doi: 10.1002/ece3.3216
Leibold M. A. (1989). Resource edibility and the effects of predators and productivity on the outcome of trophic interactions. Am. Nat. 134, 922–949. doi: 10.1086/285022
Leibold M. A., McPeek M. A. (2006). Coexistence of the niche and neutral perspectives in community ecology. Ecology 87, 1399–1410. doi: 10.1890/0012-9658(2006)87[1399:COTNAN]2.0.CO;2
Lenth V. R. (2021). emmeans: Estimated Marginal Means, aka Least-Squares Means. R package version 1.7.0. Available at: https://CRAN.R-project.org/package=emmeans.
MacArthur R., Levins R. (1964). Competition, habitat selection, and character displacement in a patchy environment. Proc. Natl. Acad. Sci. 51, 1207–1210. doi: 10.1073/pnas.51.6.1207
McPeek M. (2005). “Assembling and depleting species richness in metacommunities: Insights from ecology, population genetics, and macroevolution,” in Metacommunities: Spatial Dynamics and Ecological Communities. Eds. Holyoak M., Leibold M., Holt R. (University of Chicago Press, Chicago), 355–373.
Meyer D. J., Christiaens J., Adriaens D. (2016). Diet-induced phenotypic plasticity in European eel (Anguilla Anguilla). J. Exp. Biol. 219, 354–363. doi: 10.1242/jeb.131714
Moens T., Vincx M. (1998). On the cultivation of free-living marine and estuarine nematodes. Helgoländer Meeresunters 52, 115–139. doi: 10.1007/BF02908742
Moens T., Vincx M. (2000a). Temperature and salinity constraints on the life cycle of two brackish-water nematode species. J. Exp. Mar. Biol. Ecol. 243, 115–135. doi: 10.1016/S0022-0981(99)00113-6
Moens T., Vincx M. (2000b). Temperature, salinity and food thresholds in two brackish-water bacterivorous nematode species: Assessing niches from food absorption and respiration experiments. J. Exp. Mar. Biol. Ecol. 243, 137–154. doi: 10.1016/S0022-0981(99)00114-8
Monteiro L. C., Van Butsel J., De Meester N., Traunspurger W., Derycke S., Moens T. (2018). Differential heavy-metal sensitivity in two cryptic species of the marine nematode Litoditis marina as revealed by developmental and behavioural assays. J. Exp. Mar. Biol. Ecol. 502, 203–210. doi: 10.1016/j.jembe.2017.05.016
Newsome S. D., Tinker M. T., Gill V. A., Hoyt Z. N., Doroff A., Nichol L., et al. (2015). The interaction of intraspecific competition and habitat on individual diet specialization: A near range-wide examination of sea otters. Oecologia 178, 45–59. doi: 10.1007/s00442-015-3223-8
Parent C. E., Agashe D., Bolnick D. I. (2014). Intraspecific competition reduces niche width in experimental populations. Ecol. Evol. 4, 3978–3990. doi: 10.1002/ece3.1254
R Core Team (2023). R: A Language and Environment for Statistical Computing (Vienna, Austria: R Foundation for Statistical Computing).
Schluter D. (2000). The ecology of adaptive radiation (New York, USA: Oxford University Press). doi: 10.1093/oso/9780198505235.001.0001
Sih A., Christensen B. (2001). Optimal diet theory: When does it work, and when and why does it fail? Anim. Behav. 61, 379–390. doi: 10.1006/anbe.2000.1592
Soliveres S., Allan E. (2018). Everything you always wanted to know about intransitive competition but were afraid to ask. J. Ecol. 106, 807–814. doi: 10.1111/1365-2745.12972
Stephens D., Krebs J. (1986). Foraging Theory (Princeton, New Jersey: Princeton University Press). doi: 10.1177/1757913910379198
Stevenson I. R., Bryant D. M. (2000). Avian phenology: Climate change and constraints on breeding. Nature 406, 366–367. doi: 10.1038/35019151
Sudhaus W. (2011). Phylogenetic systematisation and catalogue of paraphyletic “Rhabditidae”. (Secernentea Nematoda). J. Nematode Morphology Systematics 14, 113–178.
Svanbäck R., Bolnick D. I. (2005). Intraspecific competition affects the strength of individual specialization: An optimal diet theory method. Evolutionary Ecol. Res. 7, 993–1012.
Svanbäck R., Bolnick D. I. (2007). Intraspecific competition drives increased resource use diversity within a natural population. Proc. R. Soc. B: Biol. Sci. 274, 839–844. doi: 10.1098/rspb.2006.0198
Svanbäck R., Pineda-Krch M., Doebeli M. (2009). Fluctuating population dynamics promotes the evolution of phenotypic plasticity. Am. Nat. 174, 176–189. doi: 10.1086/600112
Svanbäck R., Rydberg C., Leonardsson K., Englund G. (2011). Diet specialization in a fluctuating population of Saduria entomon: A consequence of resource or forager densities? Oikos 120, 848–854. doi: 10.1111/j.1600-0706.2010.18945.x
Tinker M. T., Bentall G., Estes J. A. (2008). Food limitation leads to behavioral diversification and dietary specialization in sea otters. Proc. Natl. Acad. Sci. United States America 105, 560–565. doi: 10.1073/pnas.0709263105
Toscano B. J., Gownaris N. J., Heerhartz S. M., Monaco C. J. (2016). Personality, foraging behavior and specialization: Integrating behavioral and food web ecology at the individual level. Oecologia 182, 55–69. doi: 10.1007/s00442-016-3648-8
Vafeiadou A.-M., Derycke S., Rigaux A., de Meester N., Guden R., Moens T. (2022). Microbiome differentiation of coexisting nematode species in natural microhabitats: A metagenetic analysis. Front. Mar. Sci. 9. doi: 10.3389/fmars.2022.881566
Van Valen L. (1965). Morphological variation and width of ecological niche. Am. Nat. 99, 377–390. doi: 10.1086/282379
Violle C., Enquist B. J., McGill B. J., Jiang L., Albert C. H., Hulshof C., et al. (2012). The return of the variance: Intraspecific variability in community ecology. Trends Ecol. Evol. 27, 244–252. doi: 10.1016/j.tree.2011.11.014
Visser M. E., Lessells C. M. (2001). The costs of egg production and incubation in great tits (Parus major). Proc. R. Soc. B: Biol. Sci. 268, 1271–1277. doi: 10.1098/rspb.2001.1661
Vrede T., Drakare S., Eklöv P., Hein A., Liess A., Olsson J., et al. (2011). Ecological stoichiometry of Eurasian perch - intraspecific variation due to size, habitat and diet. Oikos 120, 886–896. doi: 10.1111/j.1600-0706.2010.18939.x
Yan J., Fine J. P. (2004). Estimating equations for association structures. Stat Med. 23, 859–880. doi: 10.1002/sim.1650
Keywords: density-dependence, niche expansion, resource diversity, foraging, fitness
Citation: Guden RM, Derycke S and Moens T (2024) Resource diversity mitigates the effects of intraspecific competition in co-occurring cryptic nematode species. Front. Mar. Sci. 11:1240802. doi: 10.3389/fmars.2024.1240802
Received: 15 June 2023; Accepted: 26 February 2024;
Published: 02 April 2024.
Edited by:
Punyasloke Bhadury, Indian Institute of Science Education and Research Kolkata, IndiaReviewed by:
Tiago Jose Pereira, University of Georgia, United StatesRodrigo Riera, University of Las Palmas de Gran Canaria, Spain
Copyright © 2024 Guden, Derycke and Moens. This is an open-access article distributed under the terms of the Creative Commons Attribution License (CC BY). The use, distribution or reproduction in other forums is permitted, provided the original author(s) and the copyright owner(s) are credited and that the original publication in this journal is cited, in accordance with accepted academic practice. No use, distribution or reproduction is permitted which does not comply with these terms.
*Correspondence: Rodgee Mae Guden, rodgeemae.guden@ugent.be