Bacurú Drõa: Indigenous forest custody as an effective climate change mitigation option. A case study from Darién, Panama
- 1Department of Biology, McGill University, Montreal, QC, Canada
- 2Institute of General Ecology and Environmental Protection, Technische Universität Dresden, Tharandt, Germany
- 3Maestria Centroamaericano de Entomologia, Universidad de Panama, Panama City, Panama
- 4Division of Geological and Planetary Sciences, California Institute of Technology, Pasadena, CA, United States
- 5Tierras Colectivas Emberá del Rio Balsas, Manené, Panama
- 6Institut de Recherche sur les Forêts, Groupe de Recherche en Écologie de la MRC-Abitibi, Université du Québec en Abitibi-Témiscamingue, Amos, QC, Canada
- 7Smithsonian Tropical Research Institute, Panama City, Panama
- 8Department of Anthropology, Smithsonian National Museum of Natural History, Washington, DC, United States
- 9Département d'anthropologie, Université Laval, Quebec, QC, Canada
Efforts to naturally remove atmospheric CO2 demand that largely intact forests be maintained. Our inter-cultural research initiative tested the hypothesis that Indigenous custody of the land is compatible with the maintenance of intact forests. Here we combined traditional knowledge, phytolith analysis, remote sensing, and tree inventories to study old-growth forests in Panama's Darién. Phytoliths served to elucidate historical vegetation, remote sensing revealed the current and past Indigenous footprints while tree stature and identity characterised the forest. Until now there has been very little to no human impact within these forests and current Indigenous footprint is both small and stable. Large trees accounted for 13% of trees in the plots that we established. For over half of the species, the measured tree height was taller than previously published maximum heights, leading us to conclude that these forests are a truly exceptional ecological refugium. Noting that the local communities are not rewarded for their custody of these exceptional forests we call to revisit the Good Practice Guidance for Land Use Land Use Change and Forestry to include intact forest land. In the context of sub-optimal carbon finance options, we also propose matching as a methodology that could prove additionality of forest conservation initiatives in climate mitigation portfolios.
Introduction
As countries around the world develop their climate mitigation strategies, solutions including the agricultural, forest and land use sector have become highly popular (Griscom et al., 2017). For example, the Bonn Challenge received pledges to reforest 170 million hectares. However, efforts to naturally remove atmospheric CO2 cannot rely only on new forests regrowth but will demand that permanent, largely intact forests be maintained (Lewis et al., 2019). Primary forests cover around 27% (1.11 billion hectares) of the world's total forested area, which is estimated at 4.06 billion hectares (FAO, 2020). In that context, the critical role that Indigenous peoples play as stewards of the world's remaining forests, along with the carbon (C) stocks and biodiversity contained therein, cannot be overstated. Indigenous peoples lands account for 37% of all remaining natural lands in the world (Garnett et al., 2018) and 35% of intact forest landscapes are under traditional ownership and management (Watson et al., 2018). Globally, it is estimated that 20–22% of C stocks from tropical and sub-tropical forests are under customary control (WHRC and EDF, 2015; Frechette et al., 2018), while across Mesoamerica, including southern Mexico to Panama, estimates suggest that 49.3% are found on Indigenous lands, both legally recognized and under claim (WHRC and EDF, 2015). Furthermore, 80% of biodiversity is housed on Indigenous lands or local communities (Sobrevila, 2008; Gorenflo et al., 2012), with ecosystem services valued at hundreds of trillions of dollars (Sangha, 2020). If the international climate agenda entails conserving forests' C stocks, then ensuring the livelihoods and rights of forest-dependent people is paramount.
Yet, the potential of forests to serve as climate solutions rests on their long-term resilience and ability to withstand chronic long-term effects of climate change and other anthropogenic drivers of degradation (Ghazoul et al., 2015). The most recent report from the Intergovernmental Panel on Climate Change (IPCC) presents a new family of future climatic scenarios, coined Shared Socio-Economic pathways (SSP). The high emissions scenario (SSP 5-8.5) assumes that green-house gas emissions will remain very high in the first part of this century with a doubling in CO2 emissions between 2050 and 2100. The low emissions ones (SSP 1-1.9 and SSP 1-2.6) assume very low emissions between now and 2050 with net negative ones toward the end of the century. Global average air temperature increment under these contrasting scenarios would range from between +5.7°C and +1–1.8°C, respectively for SSP5-8.5 and SSP1-1.9-2.6. The SSP models predict that precipitations will increase with global air temperature with important regional differences. By generating climate hazards, temperature increment above 1.5°C will presence multiple risks to ecosystems and human society. Furthermore, the sixth Assessment report (AR6-WG2) asserts, with high confidence, that the predicted changes in climate will degrade and cause the loss of much of the world's forests (IPCC, 2022). The changes in climate are predicted to be “rapid… and unprecedented over centuries to thousands of years” (WG1-AR6, IPCC, 2021).
Due to human-driven land use change, deforestation and climate induced forest drought and fires, the area of primary forests is continually decreasing and becoming more fragmented (Gibson et al., 2011; Potapov et al., 2017; Mitchard, 2018). With further increase of the global surface temperature, these trends will likely continue (IPCC, 2021). Because of their connectivity, high species diversity, abundant sources of propagules and seed dispersers (Tambosi et al., 2014), intact forests, i.e., continuous forest cover over large scales (500 km2) with no remotely detectable human impact (Potapov et al., 2017), might have the ability to respond to environmental stressors in an adaptive way (Jacobs et al., 2015; Watson et al., 2018; Boulton et al., 2022). For instance, intact Amazonian forests seemed more resistant to short-term climate anomalies than human-impacted forests (Saleska et al., 2007). Esquivel-Muelbert et al. (2019) relied on 106 long-term field plots in the Amazon to document tree composition shifts in response to climate change and report an increase in seedlings of drought-tolerant species. They also pointed to the fact that, although the pace of change in forest composition lags the climatic shift, these forests show some capacity to adapt as climate in the Amazon becomes increasingly dry. There is however a void of international policies to support primary forest conservation (Mackey et al., 2020; Putz, 2020). Forests were excluded from the Kyoto protocol by the fear that a land-use focus would divert the attention away from the necessary fossil fuel's emissions reduction (Fearnside, 2001) while the focus of the Reducing Emissions from Deforestation and Forest Degradation (REDD+) mechanism is on avoiding deforestation rather than on on-going forest conservation. The concern over the fact that climate finance rewards replanting but not conserving forests is not new (see Stiglitz, 2005 in Mollicone et al., 2007). By focusing on changes in C stocks, the core principles of C accounting de facto deprived tropical countries (Streck and Scholz, 2006), and Indigenous peoples alike, of a possible source of revenues to support forest conservation.
Our case study takes place in Darién, eastern Panama. Located at the juncture between Central and South America, Darién hosts one of the last frontier forests in the world (Bryant et al., 1997; WWF, 2015). It is an integral part of the largest intact primary forest landscape of Central America (Figure 1) and amongst the most species and C rich forests in the Neotropics (Asner et al., 2013; Mateo-Vega et al., 2017, 2019). Botanically it is one of the least studied regions of the world (Kolanowska, 2015). This land-bridge, the only gap (~100 km) in the ~30,000 km-long Pan-American Highway connecting North and South America, is of global strategic importance. Completion of the Pan-American highway across the Darién gap is perceived by some as central to the continental integration of the Americas (Pereira Carneiro et al., 2019). Darién has fueled the imagination of the world for centuries. Colonial history painted the Darién as the place where Spanish explorer Vasco Núñez de Balboa became the first European to see the Pacific Ocean. Reportedly rich in gold, Darién was the first province of the Spanish Empire in the Americas (Suárez Pinzón, 2011). In 1665, the Espiritu Santo mine of Cana, Darién, was the richest gold mine in the Americas (Runk, 2015). In the nineteenth century, colonial powers, namely France, England and the US, carried out innumerable expeditions through Darién aiming to establish a transoceanic road (Suárez Pinzón, 2011; Gaviria, 2018). From these expeditions, some of them fatal, emerged a persistent representation of Darién as a rich, but dangerous and wild place (Runk, 2015). Since the 1990's, the discourse about Darién has evolved toward that of a land of violence and illicit activities with reports of illegal timber trade, destined primarily to Asian markets (Vardeman and Runk, 2020), narco-guerrillas and kidnapping. Today international coverage focuses on migrants' journeys through the “deadly” or “brutal” Darién Gap on their way to North America, where they “risk” and often lose their lives due to inclement conditions and violent thieves and human traffickers (Drost, 2020; Alcoba, 2022; Blair, 2022).
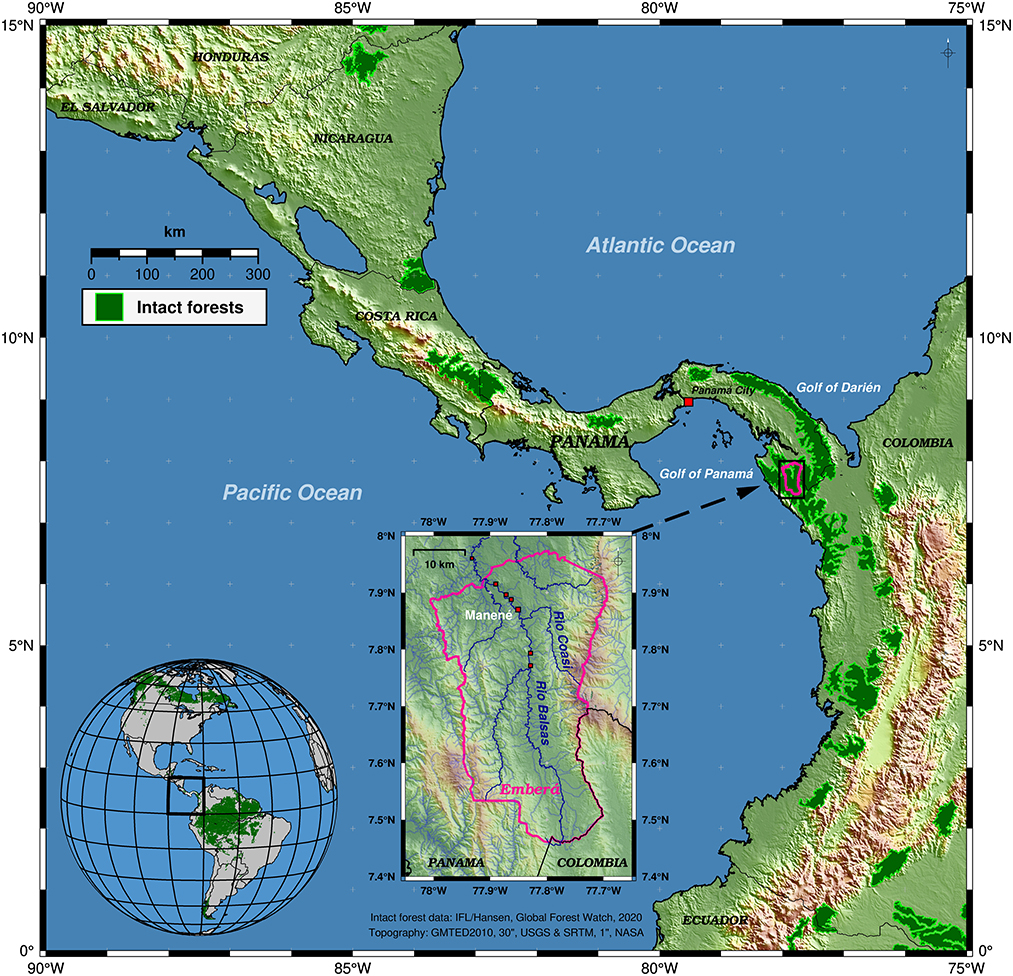
Figure 1. Overview map of the study area within the Darién region of eastern Panama and the Emberá (Tierras Colectivas del Río Balsas) territory. Green polygons highlight areas with intact forests in 2020 according to the GlobalForestWatch platform (GFW, CC BY 4.0). The main study area (inset map) is centered along the Balsas and Coasí rivers. Colored hill-shaded topography (USGS GMTED2010/NASA STRM) and borders are shown for easier map interpretation.
Darién is also an ancient bio-cultural landscape, its traditional inhabitants being the Emberá, Guna and Wounaan peoples, along with Afro-Darienitas (i.e., descendants of escaped slaves) since the early sixteenth century (Suman, 2007). Yet, past and present narratives share the common feature of playing down the presence and importance of Indigenous peoples in the region (Gaviria, 2018) indirectly suggesting that the riches of Darién are available for grab (Runk, 2015). Recent analysis of multi-commodity trafficking highlighted the fact that media reports insidiously blame Indigenous conservation efforts arguing that forests and their conservation hampers drug trafficking surveillance making the forests ideal route for illicit trading (Colectivo Darién, 2021). In 2019, the signing of a resolution to recognize Indigenous land rights within protected areas by Panamá's Minister of the Environment (Ministerio de Ambiente, 2019) opened new opportunities for Indigenous peoples. For the first time, their presence inside a protected area became legally compatible with C and biodiversity conservation. The resolution states that collective land tenure could be secured by Indigenous communities “whose boundaries overlap partially or totally with protected areas or Heritage State Forests (Patrimonio Forestal del Estado) provided that traditional occupation started before the creation of the respective protected area.” This resolution stimulated discussions with the Emberá communities and traditional authorities of the Tierras Colectivas del Río Balsas leading to the co-development of the project Bacurú Drõa (Old Growth Forests in Emberá) that seeks to establish a community-driven Old-Growth Forest Observatory.
Here we report on Bacurú Drõa's initial inter-cultural explorations of old growth intact forests of the upper Balsas River watershed in Darién, Panama, keeping in mind that the issue of paramount importance for the Emberá of Balsas River is to advance their land claim. Our main hypothesis is that the traditional presence of the Emberá on the land has been and is compatible with the maintenance of intact primary forests. To test this hypothesis, we set three objectives: (1) elucidate Indigenous presence and historical vegetation of the territory's forests; (2) reveal the current and past footprint of traditional Emberá land use over the past 35 years and (3) document the current stature and tree identity of the Balsas River forests to compare them with other well-studies sites in Panama. Results are then considered in the context of the ongoing global tropical forest deforestation crises and of sub-optimal policies and incentives to conserve intact forests thus contributing to the mounting evidence that climate policy urgently need to welcome forest conservation initiatives in its toolbox.
Materials and methods
The six Emberá communities (Pueblo Nuevo, Llano Bonito, Galilea, Manené, Bella Vista, and Buenos Aires) of the Tierras Colectivas del Río Balsas (Figures 1, 2), are remote, a 13- to−18-h dugout canoe journey from the closest town of economic importance. There is no road access and the upper river is dangerous due to a whirlpool/rapid complex. In 1980, without due consultations, Darién National Park was created by Presidential decree, engulfing the Tierras Colectivas del Río Balsas (Potvin et al., 2020). Shortly after, in 1981, the area was also named a UNESCO World Heritage site because of its rich flora and fauna including numerous endangered species (UNESCO World Heritage, 1981). Key elements, such as governance structures that legitimize land-use decisions by the Emberá, and that afford them tenure security, remain elusive given the overlapping management categories imposed on them. To the best of our knowledge no scientific expedition has ever been carried out in the region. The Emberá were interested in collaborating with scientists to inventory the biodiversity of their traditional territory and “prove to the world that they had forever conserved it” (Co-author AO).
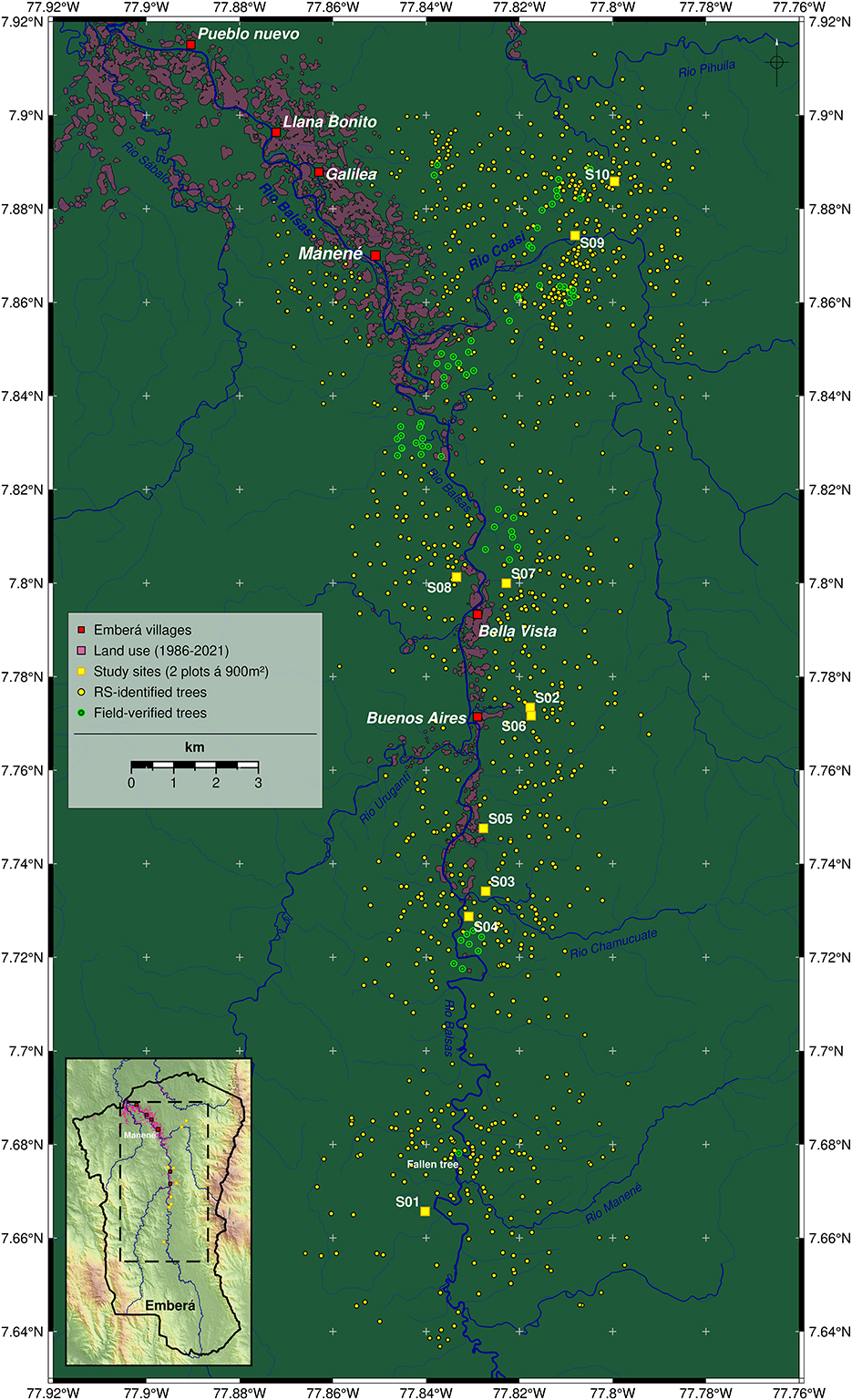
Figure 2. Detailed map of the study area. Site locations (S1–S10) are shown as yellow squares. At each site two plots of 30 m*30 m were surveyed. Further (potentially large) trees (n = 1,080), that have been identified using remote sensing (RS), are shown as yellow dots. Field-verified RS trees (n = 66) are shown as green dots. Red squares mark Emberá (Tierras Colectivas del Río Balsas) settlements. Major rivers are shown in blue. Recent past land use areas (1986–2021) are shown as semi-transparent pink polygons. Inset map shows map section (dashed line) within the border of the territory (black line) and colored hill shaded topography (NASA SRTM).
Historically, 28 meteorological stations have been established in Darién with 14 being still active (ETASA, 2022). Along the Balsas River, three stations were established, one in Manené, where it ran between 1975 and 2000. The only Balsas meteorological station currently operating is located about 60 km down river, in the floodplain with a climate very distinct from the region where we work. Lack of adequate meteorological information prevents a precise assessment of past changes in climate. The Tierras Colectivas del Río Balsas are in the North-West South America (NWS) IPCC reference region, close to South-Central America (SCA). Recent IPCC climate models for all of Central America and for NWS predict an increase in temperature with ambiguous trends for precipitation (IPCC, 2021, 2022; Castellanos et al., 2022) both regions lacking observational data and studies (Harvey et al., 2018; Sietsma et al., 2021). Overall, Central America is considered as a highly vulnerable region where climatic impact will increase the vulnerability of ecosystems and human societies although climate-change research is “notably insufficient in all sectors” (Castellanos et al., 2022, p. 1760).
A first expedition to the upper Balsas watershed in January 2019 initiated on-going field work and expeditions through 2022 by an inter-cultural team of Emberá and non-Indigenous scientists. Each scientist engaged with and trained at least two Emberá technicians. The inter-cultural team also included Emberá botanical knowledge holders. The region sampled is located between the community of Manené and the Colombian border. It is known as a drug trafficking area (Colectivo Darién, 2021) under a travel ban by most foreign embassies in Panama. Access to the zone requires approval from, and accompaniment by Panama's border police, SENAFRONT. Logistics and safety concerns limited access to sites within 5 h walking from villages.
Elucidating Indigenous presence and historical vegetation
To demonstrate that continuous Indigenous presence is compatible with the presence of intact primary forest, we relied on traditional knowledge to document the lay of the land and phytolith analysis to decipher vegetational history. Co-author MO developed Dai Ejua (Nuestro Territorio in Emberá), an Emberá map of Balsas River (Supplementary Figure S1). This handwritten map labeled the Emberá names of the streams and affluents along the river and indicated which families lived along the Río Balsas in the past. Dai Ejua served as the basis for the scientific team to gather the geographic coordinates of culturally important topograms along the river using a global position system (GPS). Topograms, through which Indigenous peoples give names to culturally important landscape features, have been proposed as a way to “write history on the landscape” (Santos-Granero, 1998). As the inter-cultural team traveled the land together, GPS point of sites of historical and/or cultural interests, such as cemeteries and topograms, were recorded together with the relevant oral history. GPS coordinates of each affluent of the Balsas River as well as their Emberá names were also recorded. This was done under McGill's Research Ethics Board Approval 21-03-023 Forest Conservation, Restoration and Livelihoods in Eastern Panama.
Phytolith analysis was carried out on soil cores from ten locations with eight sites located along 30 km of the Balsas River and two along one of its affluent, the Coasi River (Figure 2). Phytoliths effectively document vegetation from hundreds to many thousands of years into the past. These microscopic pieces of solid silica, whose shapes can be specific to various species or genera, remain intact after plants decay, serving to reconstruct historical vegetation. At each site, two soil pits 80 cm deep by 1 m wide were dug and soils were sampled every 20 cm throughout the profile. In addition, a surface “pinch” sample from the uppermost 1–2 cm of soil was taken at each location. Phytoliths were extracted from the soils by standard techniques involving sediment dispersion, removal of carbonates and organic materials, fractionation into silt- and sand-sized soil portions, and separation of phytoliths using a heavy liquid solution at a density of 2.3 (Piperno, 2006). See Supplementary material for information on the phytolith sampling and analysis.
Footprint of traditional Emberá land use (1986–2021)
To understand past and present land use in the region over the last decades, we utilized the NASA/USGS Landsat satellite archives back to 1986, retrieved via the USGS EarthExplorer service. We chose Landsat as it provides a continuous platform to go back in time with a spatial resolution of 30 m allowing to map small scale patterns of land use and forest change (Kim et al., 2014). The first villages in the Tierras Colectivas del Río Balsas were formed in the 1970s but we did not find suitable satellite imagery for this period. In total we used 18 nearly cloud-free scenes of the area between 1986 and 2021 (Supplementary Table S1). Due to the Landsat 5 and 7 detector problems (USGS, 2022) we excluded the period of 2003 to 2012 from the land use analyses. In the imagery we manually digitized bare soil areas in proximity to Emberá settlements and along the major rivers using a band combination of the near-infrared (NIR) and two short-wave-infrared (SWIR1 and SWIR2) bands (Boettinger et al., 2008). We used the appearance of bare soil within forest areas as an indicator of land use (agriculture, farming, villages). Areas of bare soil exposure within riverbeds that occur during the dry seasons were excluded. Polygon digitizing was done individually and manually (non-automated) for each scene and year using QGIS Desktop (v3.2.22). We dissolved the polygons over multiple years to avoid overlooking areas of land use within single scenes due to cloud cover or data gaps. For comparison polygons were merged and dissolved for three different time periods: 1986 to 2000, 2013 to 2021, and 1986 to 2021. While the period 1986–2021 represents the overall area of land use, e.g. recently established plantations or re-used areas, over the last 35 years, we compared an earlier (1986–2000) and recent (2013–2021) period to check whether there is an observable increase or decrease in land use and forest cover.
Current forest stature and tree identity
Forest stature and tree identity served as indicators of the value of forests for climate change mitigation. At each of the 10 sampling sites where phytoliths were analyzed, we established two 30 m × 30 m plots and measured tree height, with an ultrasonic hypsometer Haglöf Vertex IV, as well as diameter at breast height (DBH) of all trees >10 cm. Plots at each site were ~150 m apart. Hereafter, the trees measured in the 20 plots will be referred to as inventory trees (INV). As trees within a plot were measured, they were given their Emberá names by the botanical knowledge holders and a temporary unique identification number to allow scientific species identification. The later was done by examining tree architecture, characteristics of the bark and sap as well as by collecting leaves. Leaves were pressed and dried and used to corroborate species identification using the collection at the University of Panama Herbarium.
At each plot we also captured the three-dimensional (3D) forest structure using a RIEGL VZ-400i terrestrial laser scanner (TLS) with full-waveform analysis capabilities (Fa. Riegl, Horn, Austria). Each plot was scanned from at least 16 scan positions using a regular pattern and additional scans were employed to optimize coverage. Point cloud filtering and registration was performed using RISCAN Pro software (v.2.6.2). To derive canopy heights at the plot level and nearby and to extract ground points we used the LAStools (2020) LiDAR processing software. From each co-registered plot point cloud, we manually extracted individual point clouds of the largest trees (DBH > 50 cm) within the plots (n = 77, minimum of two trees per plot). Tree extraction was done using RiSCAN Pro software. DBH, tree height, and crown base height (CBH) were measured using CloudCompare (v2.11). Thereafter these measures will be referred to as terrestrial laser scanned trees (TLS). For more information on the TLS data and processing see Supplementary material.
In addition to the 20 plots, we took measurements of a large Ceiba pentandra tree that had fallen across the Balsas River (Figure 3). The tree uniquely extended horizontally over the river and hence permitted direct measurements of the length of the bole of the tree, from roots to below the branches, as well as the length of its longest primary branch. As the tree fell, secondary and tertiary branches were crushed and hence could not be measured. We measured tree diameter immediately above the buttresses as well as the height of the buttresses along the trunk. A slice of the tree trunk immediately above the buttresses was cut to estimate the age of the tree using radiocarbon dating with C14. We also scanned the large fallen C. pentandra tree using the TLS from four locations. Given the size of this fallen tree and its prominent location across the river (7.67812°N, 77.83302°W) we investigated available Sentinel-2 (European Space Agency) and PlanetScope (Planet Labs PBC) satellite imagery to estimate the date of the collapse. Multiple scenes clearly show the tree intact before and after its collapse (Figure 3), thus allowing us to determine that it fell between 20th Sept and 14th Oct 2018. We measured the fallen tree on 13th Jan 2019. The most likely explanation for its collapse is its position on a bulging slope along the riverbed that was undercut by high water during the 2018 rainy season.
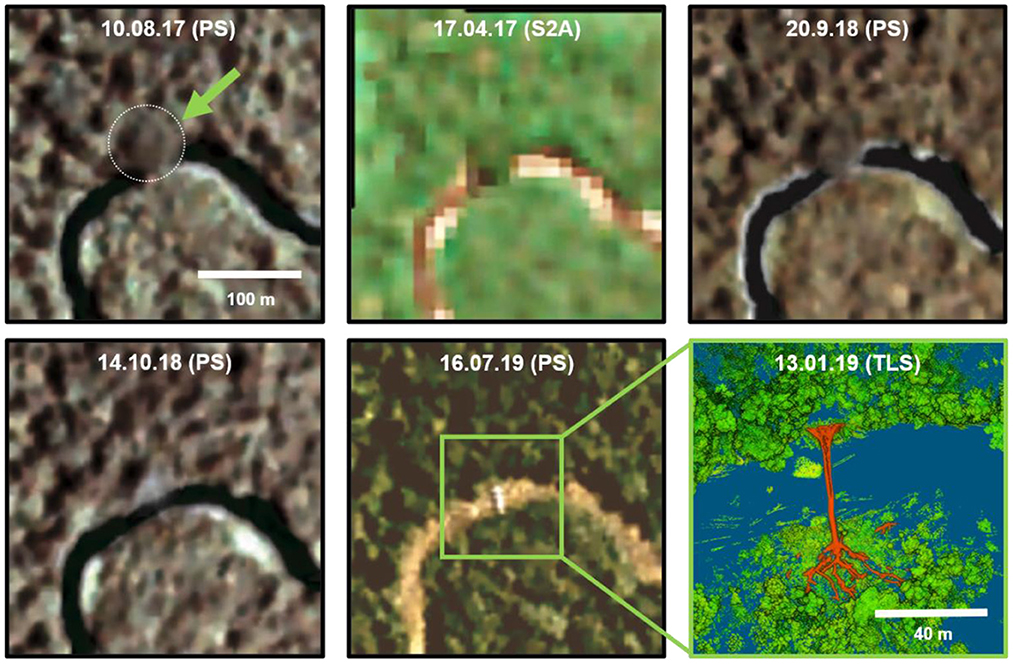
Figure 3. Spaceborne observation of a standing/fallen large Ceiba pentandra tree. Satellite images were taken from PlanetScope (PS) and Sentinel-2 (S2A) archives. Images contrast was improved for visibility. On 13 Jan 2019 the location (7.678120°N, 77.833020°W) was visited and scanned using TLS (lower right scene). The tree structure is delineated by orange color. Tree height (in the fallen state) was estimated to be >60 m, crown base height at ~40 m, and DBH above the buttress at 270 cm.
Detection of the large C. pentandra from satellite imagery prompted us to look for the presence of similar sized trees across the landscape. To do so we visually inspected 26 Sentinel-2 scenes (at 10 m spatial resolution) and 115 PlanetScope scenes (at 3 m spatial resolution) of the Balsas River basin from the years 2017 to 2021, focusing on areas that are within proximity of the study sites. The individual images were overlayed and image contrast stretching was applied to increase image contrast and feature detection. We initially marked 220 prominent potentially large trees clearly distinguishable from the surrounding forest because of a very large crown or indication of exceptional height. Trees were only marked if they were clearly identifiable in at least three scenes, one of which was a Sentinel-2 scene. The latter requirement was intended to map a specific minimum size of 2–3 pixels across, which roughly corresponds to 400–900 m2 in crown area. Sixty-six of the 220 candidate trees identified from satellite imagery were verified in different areas of the territories (Figure 2) using a GPS and their marked coordinates. Their height, DBH and species identity were recorded. Thereafter these individual trees will be referred to as remotely sensed trees (RS). After validating our ability to identify trees with large salient crowns from satellite imagery, we searched the broader landscape along the rivers Balsas and Coasi for more candidate trees (n = 860, ntotal = 1,080, Figure 2). PlanetScope and Sentinel-2 scenes were retrieved via Planet Explorer (Planet Labs PBC, 2022) and Copernicus SciHub (ESA Copernicus, 2022). Image and GIS processing as well as statistical analyses were done using QGIS Desktop (v3.22.2) and in R (v4.1.2) using the packages: raster (Hijmans, 2022), rgeos (Bivand and Rundel, 2021), rgdal (Bivand et al., 2021), sf (Pebesma, 2018) and ggplot2 (Wickham, 2016). Maps were produced with Generic Mapping Tools (Wessel et al., 2013).
We estimated the ages of six of the INV and RS tree species (n = 228) using Relative Growth Rates (RGR) of DBH measured in Panama's Barro Colorado Island (BCI) forests (Supplementary Table S5) (data downloaded from the Forest Global Earth Observatory Network; ForestGEO, 2022a). The BCI dataset included six censuses (1985–2011), typically carried out 5 years apart. We estimated the age of three common large canopy trees (Dipteryx oleifera, Anacardium excelsum and C. pentandra) as well as three common understrory trees (Sorocea affinis, Famarea occidentalis and Gustavia superba). See Supplementary material for details on RGR and age computation.
Results
Elucidating Indigenous presence and historical vegetation
We recorded the Emberá names and geographic positions of 6 communities, 10 traditional family locations prior to village settlement, and 34 geographic features (i.e., rivers, streams, headwaters, sites with cultural stories), all initially labeled on Dai Ejua. These names were agreed upon among multiple informants throughout interviews and cross-verification in distinct villages. There is consensus among the Emberá people about the names of over 30 features surrounding Río Balsas, as well as agreement over the myths explaining some of the landscape features. For example:
The river used to be blocked by a snake, or “ge” in Emberá. Behind the snake formed a large pool where the water could not flow. In those times, the river was called “ge-do,” which means “snake river” in Emberá. At some point in time, an important Jaibana (shaman) from Colombia traveled down Río Balsas, appeasing the dangerous spirits and mythical animals, that caused danger in the river. As he passed ge-do, he was able to make the snake disappear. The location then changed names from ge-do to “ge-wana,” which translates to “snake is gone.” Gewana has been the name ever since.
Such consensus provides a strong sense of place, confirming historical links between the Emberá people and their territory. Indeed, topographic writing dispersed across the landscape serves to keep memory alive and ensure the existence of current territories (Santos-Granero, 2004).
Results from the phytoliths analysis indicate low levels of historical agricultural impact on the forests. Phytoliths were common to abundant in the soils in the uppermost 40 cm below the surface (b.s.). At levels below this, very few phytoliths occurred quite possibly because the high clay content inhibited downward movement. All samples from every site that could be quantified were dominated from the bottom to the top of the profiles by phytoliths from woody forest growth, mostly trees (Figure 4). Grass phytoliths were rare to absent (0–5%) as were other plants of herbaceous, weedy growth typical of forest clearings and other human disturbances, such as Heliconia, the Asteraceae, and Cyperaceae (Figures 4, 5). The rare grass phytoliths recorded were largely from bamboos of forest growth such as Chusquea, Streptochaeta, and Guadua. Phytoliths from crop plants (maize, manioc, squashes, arrowroot, llerén) were absent. Thus, there is no evidence for significant forest clearings and the cultivation of the five commonly eaten seed and root crops listed above.
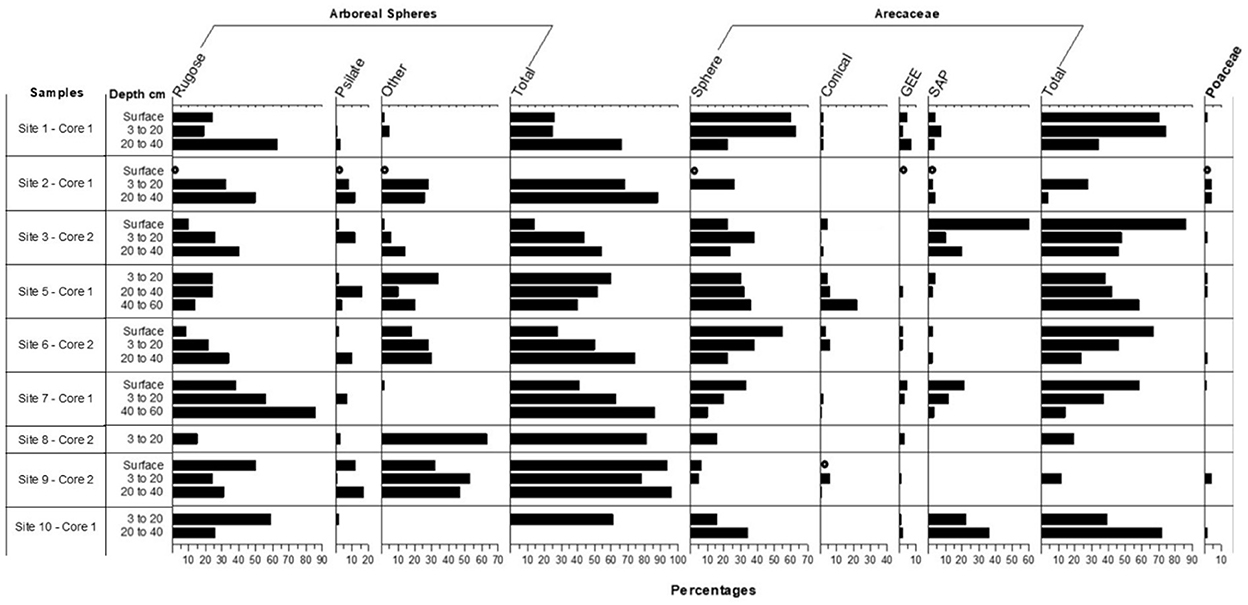
Figure 4. Percentage frequencies of phytoliths in the silt fractions of the soils at the ten sites. Where quantification occurred from 50 to 100 phytoliths were counted. For samples where counts of at least 50 phytoliths could not be achieved, taxa denoted with a circle.
Among the arboreal taxa recorded in the silt phytolith fractions are the Chrysobalanaceae, which contributed many of the rugose arboreal spheres (the genera Chrysobalanus, Hirtella, and Licania may contribute these), with the Burseraceae and a few genera in the Euphorbiaceae and Moraceae contributing fewer of those forms (Figure 4). Palm phytoliths were recorded in significant numbers (Figure 4). It is noteworthy that some of the tree genera identified in the phytolith record were also sampled in the current vegetation in particular the genera Licania, Guatteria, Oxandra and Brosimum. Similarly, in current vegetation, Moraceae is the most abundant family in 4 of the 10 studied sites while the Arecaceae is the dominant family in three of the sites. Present in the sand phytolith fractions were tree phytoliths from Talauma, a genus not found in the present vegetation, and genera in the Annonaceae (Guatteria and Unonopsis and/or Oxandra) (Figure 5). Celtis phytoliths (Figure 5) may be from tree or liana members of the genus. Arboreal Elongate and Baculate phytoliths may derive from a small number of unrelated genera of trees, shrubs, and vines (Protium—Burseraceae, Licania, Hirtella, Brosimum—Moraceae, and Mendoncia—Acanthaceae).
Palm genera such as Oenocarpus and Euterpe produce identifiable phytoliths (forms called SAP under Arecaceae in Figure 4 indicating the spheroidal type with short acute projections) (see Morcote-Ríos et al., 2016; Piperno et al., 2021; Witteveen et al., 2022). Looking at the Arecaceae percentages in Figure 5, there is an increase in the Oenocarpus/Euterpe (SAP) phytoliths at five sites—1, 2, 3, 6, and 7—occurring either at the surface or beginning at 3–20 cm below the surface. These phytoliths increase dramatically to 60% of the count in the surface sample from Site 3 indicating the vegetation there saw a large increase of one or both genera probably in the past few hundred years. At Site 7, these phytoliths also increase significantly from 3 to 12% from 60 to 3 cm b.s., and to 21% in the surface sample. Judging from directly dated phytoliths from other terrestrial soil profiles (e.g., McMichael et al., 2012; Piperno et al., 2015, 2021), the increase to 12% at 3–20 cm b.s. likely occurred during the past 2,000 to 3,000 years. The other types of palms phytoliths that increased in frequency at Sites 1, 6, and 7 (category Sphere under Arecaceae in Figure 4) may have derived from several genera including Attalea, Elaeis, Manicaria, and Phytelephas. The increased abundance of palms on the landscape may have resulted from human activities during the past few thousand years. The conical type of palm phytoliths that show no increase through time may have derived from genera such as Astrocaryum, Bactris, Socratea, or Iriartea. They generally occurred in low or absent frequencies.
Data on current vegetation echoes some of the phytoliths results. O. mapora is a frequent palm at the sites today whereas Euterpe spp. are not, and it is thus likely O. mapora is one of the palms represented in the phytolith samples studied here. Current vegetation surveys showed surprisingly high abundance of two palm species, O. mapora at site 1 and Iriartea deltoidei at site 8. At site 1 O. mapora represents 36% of the stems while at site 8, I. deltoidei represents 50% of the stems. Out of the 20 vegetation plots surveyed, 4 showed higher palm density than others with an average of 35.4% of palms out of total stems. The average percentage of palms for the remaining 16 plots was 5.6%. This result indeed suggests that in some sites palms were enriched by the Emberá—a traditional form of “forest caring.”
Most of the localities did not see fires of enough intensity to leave evidence in the form of charcoal and burned phytoliths. Small pieces of macroscopic charcoal were recovered from four sites; Site 3 (at 3–20 cm b.s), 7 (from 3 to 40 cm b.s.), 8 (55–60 cm), and 11 (20–40 cm). Rugose and psilate arboreal spheres also showed considerable evidence of burning in levels where Oenocarpus/Euterpe increased. For example, the highest levels of burned phytoliths −38%—all from arboreal rugose and psilate spheres, were also found at site 7 from 3 to 25 cm b.s. At other sites, burned phytoliths were largely absent except for Site 3, where 11% of the palm phytoliths were burned. Because tropical forests today infrequently burn from natural causes, the data indicate that human activities caused the increases in fires and palms. However, neither phytolith nor charcoal records indicate past occurrence of slash and burn agriculture or significant forest clearing of any kind leading to areas invaded by weedy herbaceous growth.
Footprint of traditional Emberá land use (1986–2021)
The Landsat satellite imagery showed that the total area of man-made bare soil exposure between 1986 to 2021 was 1,655.99 hectares, which corresponds to 1.3% of the total Emberá territory (area of land claimed is 127,533.3 hectares—Figure 2). Non-forest land cover is concentrated at lower elevations, below 105 m, and on flat terrain along the rivers Balsas, Urugandi and Pihuila (Figure 6). Although the land use is highly fragmented, there is a clear pattern of repeated and frequent re-use. Looking at the early period from 1986 to 2000 land use through human intervention totaled at 1,229.37 hectares. For the later period, between 2013 and 2021, we mapped a land use of 707.28 hectares, mainly on the northern and eastern parts along Balsas River. Deforestation remained generally within 1 km from any major river. In very rare cases changes in forest cover can be observed up to 1.5 km away from rivers, but only when the terrain is widely flat and with slopes of <7°. There are small-scale areas at which an increase in land use is noticeable, for example around the village of Bella Vista, a community that was founded only a few years ago.
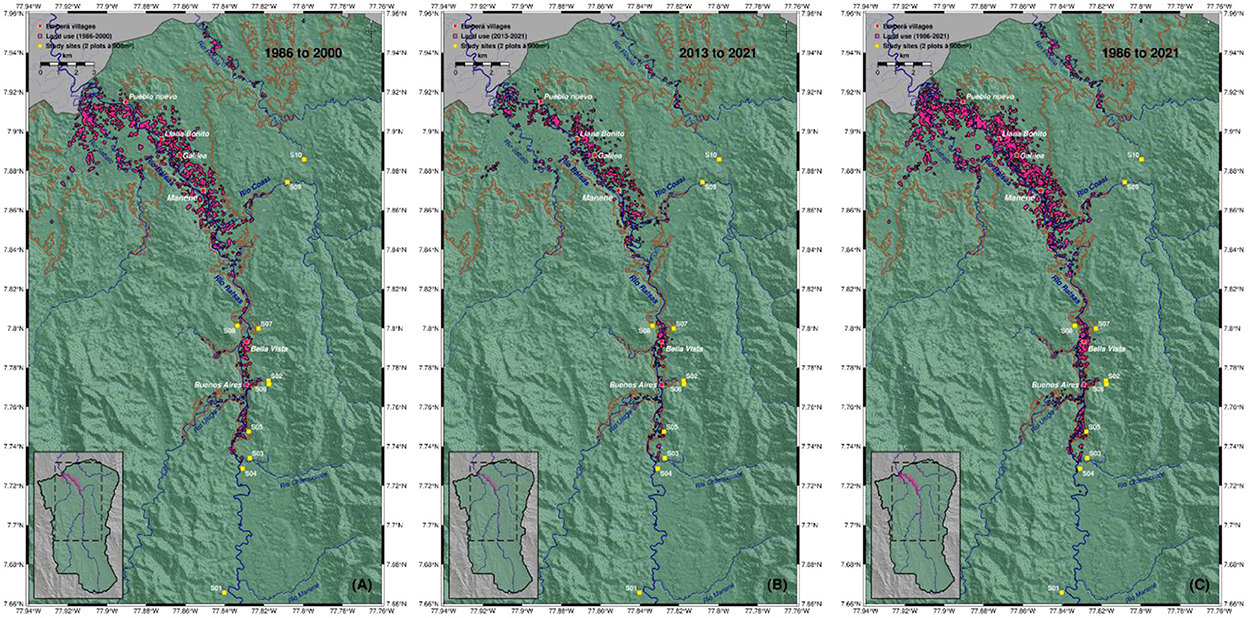
Figure 6. Areas of identifiably bare soil exposure (as an indicator of land use; pink polygons) in the Emberá (Tierras Colectivas del Rio Balsas) territory since 1986. Individual maps show different time periods [(A) 1986–2000, (B) 2013–2021, (C) 1986–2021] to indicate that areas are frequently reused and mainly bounded to flat terrain below 105 m elevation (brown line). Mapping is based on Landsat (4-TM, 5-TM, 7-ETM, 8-OLI) satellite scenes. Also shown are Emberá villages (red squares) and study sites (yellow squares). Background shows hillshaded topography (NASA STRM). Inset map shows the map section (dashed line) within the full territory (green color).
For comparison we investigated the Global Forest Watch (GFW) dataset (Hansen et al., 2013) that maps annual forest change (gains and losses) on an automated basis. GFW reveals a trend of forest loss near settlements and along rivers. However, the GFW baseline starts with the year 2000 whereas our analyses go back 15 additional years. The discrepancy could be explained by the fact that old-fallows and secondary forests are re-used over a longer time then the 20 years of GFW analysis. In the GFW data changes in forest areas might be flagged as “loss” while in reality it is a form of “re-use” with no loss of primary forests. Overall, we found no evidence of large-scale expansion in deforestation or parcel sizes in the Emberá territory, and our analysis suggests that land use has remained stable since the late 1980s.
Current forest stature and tree identity
In the 20 inventory plots we measured height and diameter for 640 trees. Ten trees were taller than 60 m and 36 other trees between 50 m and 60 m in height (Figure 7). The laser scanning data confirmed the high canopy with ten of the 20 plots showing a maximum TLS canopy height taller than 50 m (Figure 8). Plot 17 at site 9 towered over the rest at 56.5 m. Plots 03, 05, 08, 16, and 17 likewise show tall canopies. Median TLS canopy heights within 30 m radii around the plots ranged from 27 m at site 2 to 46 m at site 9 (Supplementary Table S2). Comparing the height of the 78 large trees extracted from the TLS data showed that the INV-height were significantly higher by on average 10.8% (W = 3,903.5; p < 0.05) and than the TLS-height (TLS height = 39.8 m ± 7.3 m; INV-height = 44.6 m ± 12.3 m) (Figure 7). The manual measurement of DBH showed 44 trees above 100 cm with an additional 52 trees with ≥50 to <100 cm DBH (Figure 7). The sheer size of individual trees in terms of DBH is also impressive, with sites 3-4-5-7-9 having trees with DBH ≥ 200 cm. The 10 sites sampled differ in the prevalence of large trees (i.e., trees with DBH ≥ 50 cm) ranging between 2 at site 8, and 14 at site 5. DBH measured on the TLS-extracted large trees was not significantly different from the INV measurements of the same trees (W = 5,380.5; p > 0.05).
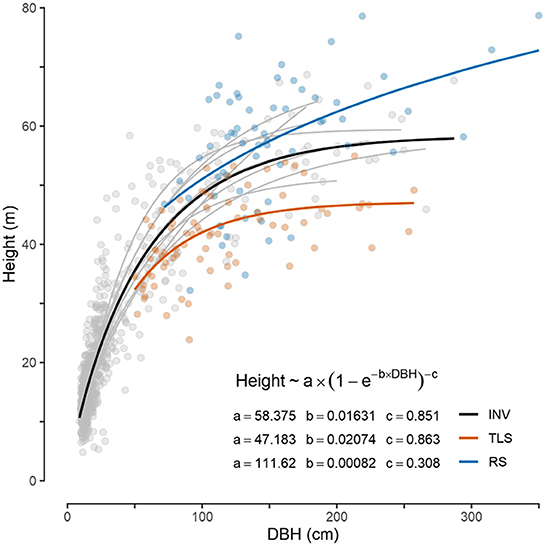
Figure 7. Scatter plot of tree height versus diameter at breast height (DBH) for the three groups of trees that were measured: INV, conventional tree inventories in 10 sites across the landscape; TLS, individual extracted trees from terrestrial laser scanning data of the inventory plots; RS, field-verified trees that have been identified by remote sensing. Colored lines show a non-linear least-squares regression fit (Richards growth function) for each group to indicate the overall tendency of the relationship. Parameter estimates are given next to the legend (initial guesses were set to a = 75, b = 0.01, c = 1). Thin gray lines show fit for INV data at individual sites.
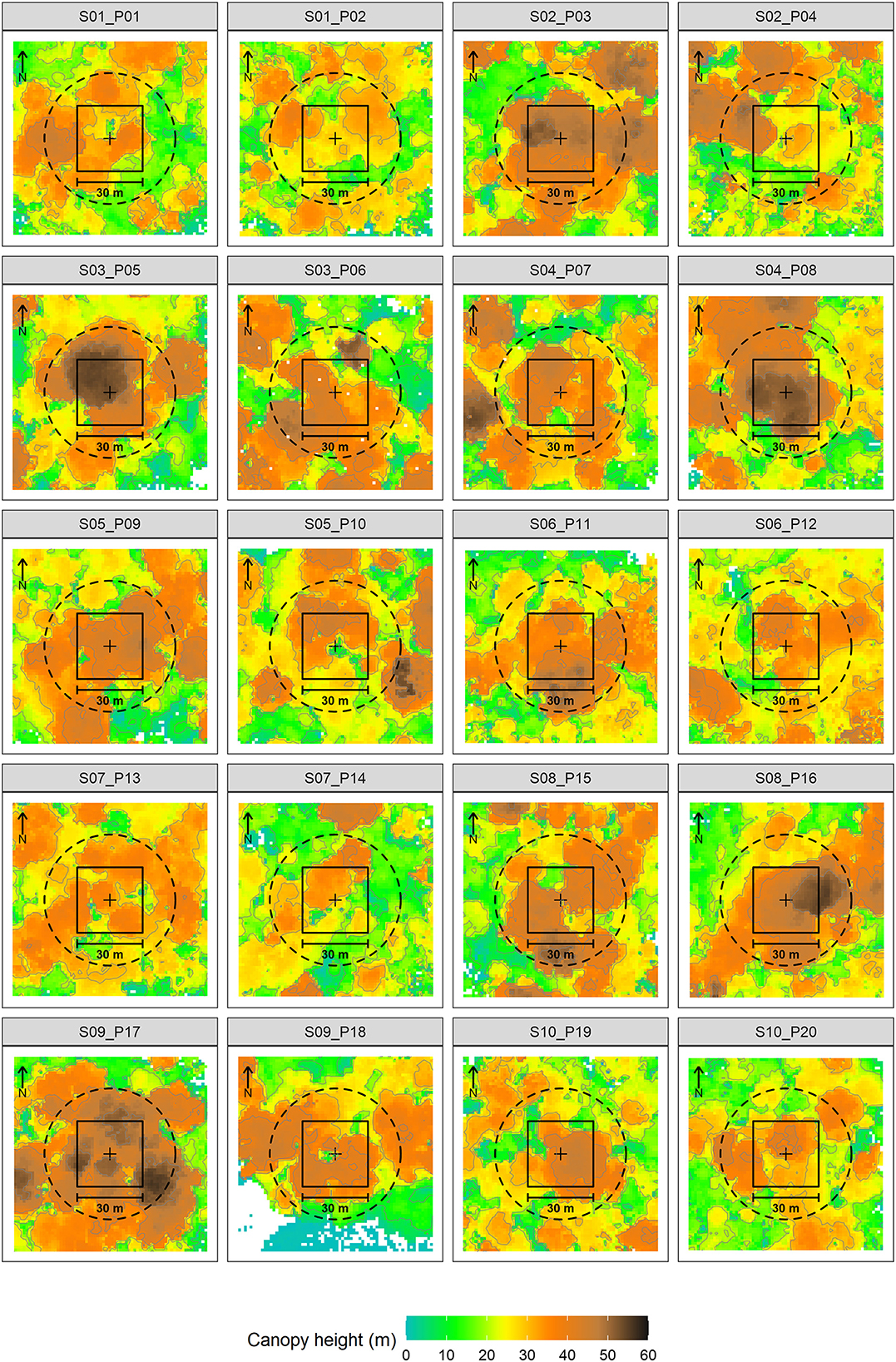
Figure 8. Color-coded canopy heights at TLS captured sites. Black line marks the 30 m*30 m plot boundary, dashed line marks a 30 m radius around the plot center (black cross). Site and plot numbers are given in the heading.
We identified 70.8% of the INV trees to the species level, 13.3% to the genus, 3.3% to the family level, and 12.5% to morphospecies. In the 1.8 hectares sampled by our inventory plots, we found 120 species or morphospecies spread over 104 genera and 33 families. The study sites with the highest density of individuals recorded were site 6 with 94 individuals in 1,800 m2, followed by site 8 with 90 individuals and site 1 with 77 individuals. Among the families with the highest abundance of species, Fabaceae stands out with 25 species, followed by Moraceae with 12 species, Malvaceae with 10 species, Arecaceae with 6 and finally Lauraceae and Rubiaceae with 5 species (Table 1). The most common tree species are: Sorocea affinis with 53 individuals and with presence in all 10 sites followed by Faramea occidentalis with 40 individuals found in 7 sites and Guatteria lutens with 30 individuals found in all 10 study sites.
We compared the height of the INV trees to the maximum height (MaxH) reported by Condit et al. (2020) in their comprehensive assessment of the trees of Panama (Figure 9). For over half of the species, INV height measured in Darién was taller than previous published MaxH. This is the case for both large statured trees and smaller ones including two of the most abundant species. Prior to this scientific expedition F. occidentalis was reported to grow up to 9 m, yet we measured 35 individuals taller than 10 m and 8. Similarly, for S. affinis the literature reports a MaxH of 20 m. The tallest S. affinis tree in the plots measured 41 m and 26 individuals were taller than 20 m. A clear characteristic of the Balsas forests is its previously undocumented height.
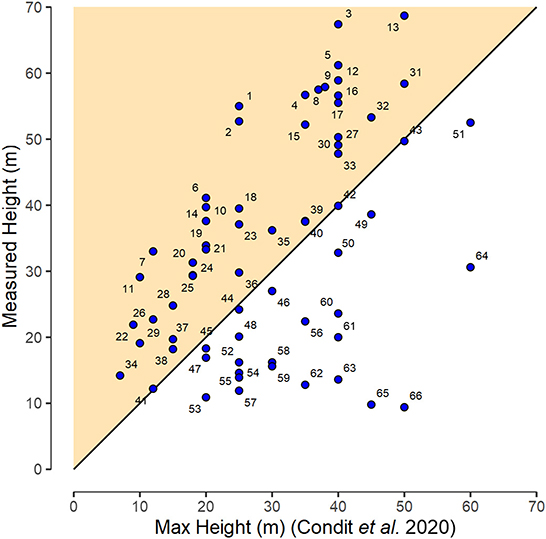
Figure 9. Comparison of manually measured tree heights (INV) for different species with that reported in the literature as the MaxH by Condit et al. (2020). For figure clarity species are coded as individual numbers (1–66). Please refer to Supplementary Table S3 for respective species names. The colored triangle indicates species that exceed the previously reported MaxH. Black line is the 1:1 line.
Across the 20 inventory plots, very large trees (DBH ≥ 150 cm) belong to 11 species with A. excelsum (nine individuals) and D. oleifera (six individuals) being the most frequent. Other species achieving very large diameters were Carapa guianensis, Apeiba membranacea, Ceiba pentandra, Clarisia biflora, Dilodendron costaricense, Guatteria cf allenii, Luhea seemanii, Myroxylon balsamum and Vatairea erythrocarpa. In contrast, the diversity of the 66 RS trees identified by their large crown is reduced and showed a large dominance of D. oleifera that was represented by 41 individuals followed by C. pentandra with 8 individuals, V. erythrocarpa with 5 and A. excelsum with three individuals. It is noteworthy that D. oleifera is found in the Convention on International Trade in Endangered Species of Wild Fauna and Flora (CITES) Appendix 3. Six other species across size classes have a known conservation status (Table 2). Vitex masoniana, one of the two endangered species that we sampled, was found in 5 of the 10 sites sampled. Likewise, Brosimum utile and Tocoyona pittieri, both classified as vulnerable by IUCN, have a good distribution across sites (Table 2).
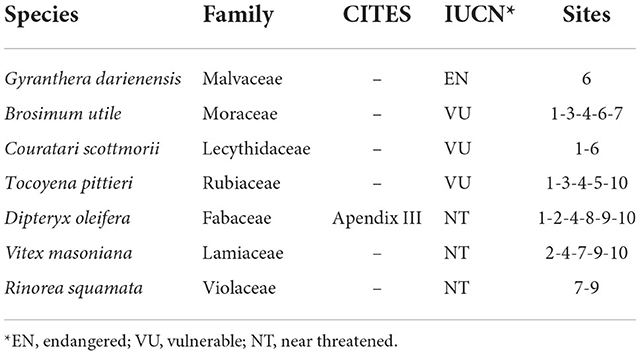
Table 2. Conservation status of threatened species encountered using both CITES Appendix II (https://cites.org/eng/app/appendices.php, accessed 15.08.2022) and IUCN lists.
The size of the fallen C. pentandra tree and remote sensing analyses further informs us of the stature of trees from the Balsas forests. From roots to branches, the bole measured 39 m and the longest primary branch added another 31 m to the tree suggesting that when standing the tree was over 70 m tall. Its DBH immediately above the buttresses was 270 cm. The TLS scans estimated tree height as at least 61 m with a crown base height of almost 40 m (39.6 m). The crown width was estimated at 40 m diameter from TLS data and satellite imagery (Figure 3), corresponding to a crown projection area of 1,257 m2, assuming a circular crown shape. Likewise, the 66 RS trees showed a mean height of 57.1 m and a mean DBH of 152.7 cm (Figure 10). Theses field-validated trees grow at elevations between 100 and 250 m and slopes of up to 20° (Supplementary Figure S2). Having confirmed our ability to detect large trees using satellite imagery, a thorough search across the landscape identified 1,080 locations of prominent potentially large trees along the Balsas and Coasi Rivers (Figure 2). These data provide strong evidence that these exceptionally large trees (height > 50 m, DBH > 150 cm, CPA > 700 m2) are common in the studied area.
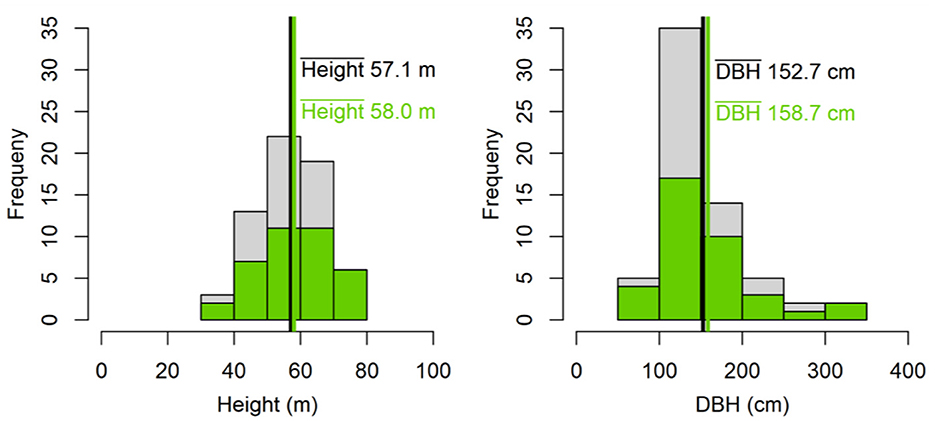
Figure 10. Histogram and mean values of tree height and DBH of all RS trees (n = 66) that have been identified using remote sensing and validated by field measurements. Green color shows histogram of trees within proximity (3 km buffer) of the 10 sites (n = 37).
Finally, we estimated tree age for 228 individual trees of six different canopy and understory species with median estimated age of 368 years. Based on their size and BCI Median RGR, G. superba trees would include the oldest specimens found in Balsas ranging 567–1,046 years while the three canopy species, A. excelsum, D. oleifera and C. pentandra, reached respectively 696, 677, and 478 years (Figure 11). The C14 age estimate obtained for the fallen C. pentandra dated it 207 years, coherent with the 95% lower confidence interval age that we estimated.
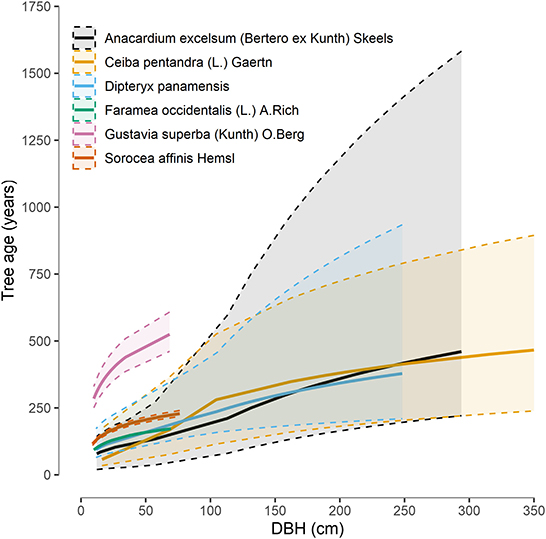
Figure 11. Age estimates by diameter at breast height (DBH) for seven species in our study based on their recorded relative growth rates (RGR) on Barro Colorado Island. Means (thick lines) and 95% confidence intervals (dashed lines) were derived from 228 trees (RS + INV).
Discussion
Balsas: A bio-cultural landscape preserving intact primary forests
Emberá stewardship of the land has maintained intact old-growth forest throughout much of their 125,000 hectares. Indeed, land cover analyses showed that since 1986 the footprint of the communities in the Tierras Colectivas del Río Balsas impacted only 1.3% of the entire territory, and that the coverage of agricultural land area has remained stable over the past 35 years. With around 100 households scattered across the Tierras Colectivas del Balsas, observed footprints of ~1.6 hectares per household fall in the low range of published values of 1.5–16 hectares per household, which contrasts with colonists' household footprints of 8 to 33 hectares (Gray and Bilsborrow, 2020). Our results are similar to those from other studies showing that Indigenous or local community stewardship with functioning governance structures can support the conservation of nature and improved livelihoods of people (Kothari et al., 2014). For example, in the Northern Ecuadorian and Peruvian Amazon, Indigenous' and local communities' household footprints have remained both low and stable through time (Gray and Bilsborrow, 2020; Coomes et al., 2022). The Peruvian study was based on 275 communities and analyzed forest cover between 1989 to 2015 while the Ecuadorian study analyzed 500 households between 2001 to 2012 revealing a very low rate of primary forest clearing, which was mostly restricted in time to initial settlement, largely because local people prefer cultivating secondary growth forests (Coomes et al., 2022). Likewise, the land management approaches currently employed by the inhabitants of Tierras Colectivas del Río Balsas allow sustaining the Emberá people while being highly successful at conserving the old-growth intact forests and their large C sinks.
Until the 1960's the Emberá report living as dispersed family groups mostly located at the convergence of the Balsas River and its affluents. Traditional Emberá households have three zones of land extending perpendicular to the river (Bennett, 1968). The zone adjacent to the river was 50–75 m wide and contained the house site, animal pens, plantain groves, and gardens. The second zone was generally <1 km wide and contained swamplands and monsoon forest with pigs, wild plants, and game animals. The third zone, extending several kilometers into the forest, contained regrowth vegetation, was used for grain and tuber cultivation. This traditional subsistence pattern has been modified over time as settled Emberá villages formed beginning in 1962 (Supplementary Table S4; Herlihy, 1986). In villages, households are clustered in a large area of cleared natural vegetation generally extending more than 100 m perpendicular to the river (Herlihy, 1985). The villages are surrounded by a zone where native forest is typically cut for 3–6 km or more and cultivated in small patches. Since villages were formed, subsistence activities, including cultivation of fruit trees, banana plantations, hunting and collecting activities, are “located at considerable distance from domestic sites” (Herlihy, 1985).
With the phytolith data, we show that forests even close to villages have never been subjected to large scale cutting, burning nor agriculture. The only other palaeoecological studies of Darién took place near the archeological site of Cana (Bush et al., 1992; Piperno, 1994). It provided strong evidence for 4,000 years of pre-Colombian indigenous occupation and agriculture with maize cultivation playing a central role. Maize cultivation was associated with grass burning (Piperno, 1994) and likely sustained a relatively high human population. Analysis of charcoal and phytoliths similarly unveiled the presence of important agricultural centers in the Amazon basin (Bush et al., 2015). However, even in these relatively densely populated archeological landscapes, the presence of humans at distances >15 km from such centers was trivial. There are two plausible explanations regarding the difference in phytolith record from Cana and Balsas. At the arrival of the Spaniards, inhabitants of Panama and Colombia were skilled gold smithers as evidenced by rich museum collections in both countries (Banrepcultural, 2022). The riches of Cana might have attracted a pre-Colombian population larger than in other parts of Darién. Topography might further explain the different historical settlement patterns between the two locations. The upper Balsas River is surrounded by mountain chains with Pirre (1,615 m) and Altos de Nique (1,730 m) on the North and the Jurado and Baudo Mountain chains on the South. Conversely, the Tuira river, which gives access to the Cana valley opens on the Atrato river plain in Colombia. The passage from the Tuira river to the Atrato river has been identified by the Spaniards at the end of the seventeenth century as the best route to South America (Suárez Pinzón, 2011). Undoubtedly, the pre-Colombian inhabitants of the region had likewise identified this natural passage.
Important forms of cultural forest use in the tropics involve the care and possible enrichment of native perennial tree species. Some of these economically important species, such as the avocado (Persea americana), cacao (Theobroma cacao), hogplum (Spondias mombin), soursop (Annona muricata), other Annona species, and cashew (Anacardium occidentale), are “silent” in phytolith records due to a lack of phytolith production or absence of diagnostic phytoliths. However, critical and readily detected economic taxa include palms, that are prolific phytolith producers in all structures of the plant. Palm genera such as Oenocarpus and Euterpe have been shown to be of high economic importance in the past as they are today (Morcote-Ríos et al., 2016; Piperno et al., 2021; Witteveen et al., 2022). Traditional practices of land cultivation related to palms have been documented among various Indigenous peoples. For the Kayapó, palms predominate in the inventory of plants in managed forest plots because they offer a variety of uses (Posey, 1985). The Chachi Indigenous group in northwestern Ecuador also historically utilizes nine palm species for 38 different uses, including food, utensils/tools, and construction (Schneider et al., 2017). In fact, in tropical environments, palms are considered the third most important plant family, following grasses and legumes (Johnson and IUCN/SSC Palm Specialist Group, 1996). The strongest evidence that we found of past human interventions in the Balsas forests was an increase in palms phytoliths in four of the 10 sites at different soil depths and moments in time, as well as a high density of the two useful palm species, namely O. mapora and I. deltoidei, in the present vegetation. Levis et al. (2018) identified eight different practices of Indigenous peoples in Amazonia that result in increased density of useful plants: removal of non-useful plants, protection of useful plants, attraction of non-human animal dispersers, transportation of useful plants, selection of phenotypes, fire management, planting of useful plants, and soil improvement. Currently in the Tierras Colectivas del Río Balsas, several of these practices are still in use. For example, O. mapora, called sokarjó in Emberá and trupa in Spanish (Herlihy, 1986), has been historically used by the Emberá people (Johnson and IUCN/SSC Palm Specialist Group, 1996). The nutritious flesh of the fruit contains oil and good-quality protein comparable to animal protein (Henderson et al., 1995). The high density of O. mapora Mart observed at site 1, unusual from a botanical standpoint, strongly suggests intentional human care. An interview with a villager born at site 1 provided the explanation for the high abundance of sokarjó palms. She indicated that her father tended the palms in the area, confirming the existence of some form of Emberá “cultivation.” Co-author FS also mentioned the existence of a sokarjó resguardo (refuge) near one of the Balsas River villages where it is not permitted to fell the forest nor the palm to ensure its long-term availability.
Characteristics of Balsas's old-growth forests
A LiDAR-derived C map for Panama identified Darién as the region with the highest C stocks (Asner et al., 2013) and the results of our field campaign confirm that indeed the forests of Tierras Colectivas del Río Balsas are of exceptional stature. We contend that the history of Indigenous land stewardship by the Emberá's of Tierras Colectivas del Río Balsas is a key determinant for the continued existence of these unique forests. Characterizing forest structure demands large area because of the irregular distribution of large trees on the landscape (Lutz et al., 2018). Using global data from 48 large permanent forest plots, these authors estimated that 6.5 hectares of forests was necessary to estimate the biomass of one plot because of their spatially heterogeneous distribution. Although the area of our inventory plots is small the joined remote-sensing analysis of trees with large crowns at the landscape level yield data that are sufficient to capture key characteristics of these old-growth forests. For comparative purposes, scaling the average density of trees ≥50 cm (9.7) per plot to one hectare yield 54 trees compared with 36 in the five undisturbed 1-hectare forest plots sampled by Mateo-Vega et al. (2019), that were located lower in the Balsas River watershed. Harris et al. (2021) used 13 plots of 10 hectares to estimate the number of trees with DBH ≥ 80 cm in a forest of the Republic of Congo. On average, 94 large trees were found in 10 hectares. Our sampling in the upper Balsas territory included 57 trees of the same stature in 1.8 hectares, suggesting a density of large trees per hectare 5.5 time bigger. In the eastern Amazon trees with DBH > 60 cm represented 9.3% of the stems, while in La Selva Costa Rica, they accounted for 2.5% of the stems. In the forest plots that we established along the Balsas River in Darién such large trees accounted for 13% of the individuals. A pan-tropical study reported the density of trees ≥70 cm DBH at 7.5 ± 5.3 trees per hectare across South America (Slik et al., 2013). In the total area that we sampled in Balsas (i.e., 1.8 hectares), we encountered 71 trees >70 cm DBH, equivalent to 5.2 times more large trees per hectare.
Several studies have shown strong correlations between the density of large trees and forests' aboveground biomass (AGB), hence C stocks (Sist et al., 2014; Forrester, 2021). AGB computed from the 20 largest trees in a hectare of tropical forest provide an unbiased estimate of AGB, albeit with 17.7% of relative error (Bastin et al., 2018). Surveying over 600,000 trees in 400 locations, Stephenson et al. (2014) showed that trees with 100 cm DBH add three times more biomass per year than trees with 50 cm DBH. Large trees not only play a crucial role in determining C stocks but also timber yield and forest structure, as they sustain biodiversity and act as drivers of hydrological cycles (Forrester, 2021; Jucker et al., 2022), yet are globally endangered (Lindenmayer et al., 2012). The study of large trees is challenging because of their low density and longevity (Sheil et al., 2017; Wang and Ali, 2021).
In Amazonia, airborne LiDAR has been used to identify trees taller than 50 m in 540 transects of 375 hectares for a total area of 202,500 hectares, with the tallest trees reaching 80 m (Gorgens et al., 2021). In nearby upland Chocó forests of Colombia, mean canopy height measured by airborne LiDAR was 21.8 m (Meyer et al., 2019). We recorded a mean TLS-plot height of 33.0 m within 30 m around the plot centers and directly measured 52 trees taller than 50 m in 1.8 hectares and 11 taller than 60 m in the 20 inventory plots. The RS trees in our study further suggest that large trees are common across the Balsas River landscape. Interestingly, we report tree height taller than previously reported MaxH (Condit et al., 2020) for 40 species including canopy and understory species. F. occidentalis (L.) A. Rich., one of the most abundant species in the 50-hectare permanent plot of BCI, has a reported maximum height of 9 m taller than 20 m. The tallest D. oleifera, an important canopy tree, reached 68 m. There is a well-known trade-off between functional traits that enhance fast growth vs. longevity, with fast-growing trees having lower wood density than slow growers (King et al., 2006; Rüger et al., 2020). In Balsas however, the species that grew taller than their reported MaxH varied in wood density between a low of 0.2669 g cm−3 for I. deltoides to a high of 0.867 g cm−3 for Astronium gravolens. Thus, species with different life strategies showed the same pattern. A possible explanation for the exceptional height of the trees is their age. Both our direct age estimate for the fallen C. pentandra and the indirect age estimates based on BCI's RGR show that trees are vastly older than 100 years and can thus be considered as old trees (Ali and Wang, 2021).
Comparison of tree identity for the Tierras Colectivas del Río Balsas with BCI and San Lorenzo, two sites where ForestGEO operates permanent plots in Panama, points to important differences in forest composition. BCI is a tropical moist deciduous forest receiving on average 2,580 mm rain per year while San Lorenzo receives 3,000 mm per year. Based on data from Camoganti, the nearest operating meteorological station to the Tierras Colectivas del Río Balsas, average annual precipitation of 2,741 mm was recorded for the period between 2000 and 2014. Yet of the 120 tree species or morphospecies identified, 68 are absent from the forests of BCI and/or San Lorenzo. The Balsas forests shares 17 species with BCI and five species with San Lorenzo. A comprehensive study of 56 study sites in 10 countries across the neotropics showed that during succession, secondary forests recover species diversity relatively rapidly with 90% of the species richness of old-growth forests recovered in 31 years (Rozendaal et al., 2019). Species composition, however, does not recover as rapidly, with an estimate of 780 years to recover the full species composition of a pristine forest. According to Rozendaal et al. (2019), forest composition recovery time depends on the size of the regional species pool, with diverse tropical forests taking longer to recover completely. The forests of both BCI and San Lorenzo are located along the historical “Camino de cruces” that the Spaniards used for the transit the gold of Peru and where the Panama Canal is located. It is therefore likely that these forests have been disturbed in the past and are now on a slow path to recover species composition, which could explain the differences with the primary forests of the Balsas River. The long timeline to composition recovery makes primary forests such as those we studied essentially irreplaceable, supporting calls to recognize them as of global concern (Mackey et al., 2015).
Environmental conditions could also in part explain why the Balsas forests are exceptionally developed. Across the tropics, the density of large trees tends to increase with soil fertility and rainfall (Slik et al., 2013). The study of tree height in the Amazon showed that the 70–80 m very tall trees were concentrated in the eastern Amazon with the best explanatory variable being the number of clear days per year and the fraction of soil clay content (Gorgens et al., 2021). In Costa Rica, soil type was also a determinant for the presence of large trees (Clark et al., 2019). We showed that mean annual cloud cover frequency for 2000–2014 for the Tierras Colectivas del Río Balsas was well above 80% (Supplementary Figure S4) indicating a very stable cloud cover throughout the year (Wilson and Jetz, 2016) while the soil that we collected for the phytolith analysis were very clayish (Supplementary Figure S5). Maps of soil texture for Panama (IDIAP, 2010) also reveal that soils in the Balsas River area are comprised primarily of clay loams, with very high clay content. We propose that the large density of large trees in Balsas is partially explained by a combination of old age, favorable climatic conditions, and soil types that are conducive, as in other parts of the Neotropics, to enhanced tree growth. These unique characteristics of the Balsas forests makes them a truly exceptional ecological refugium sensu Mackey et al. (2012).
Due to a lack of long-term observations and weather stations in the region, it is unclear whether the favorable climatic conditions will persist under climate change. Interpolation modeling based on meteorological data from Darién show a declining trend in annual precipitation between 2005 and 2015 (Supplementary Figure S7) (Varadarajan et al., 2022). Such trend is coherent with SSP scenarios for nearby SCA region, where increased drought is predicted, but not for the NWS one (Kusunoki et al., 2019; IPCC, 2021). Indeed future climate scenarios for NWS predict both an increase in mean temperature and in median precipitation (Supplementary Table S6), the confidence for these trends being higher for temperature than for precipitations (IPCC, 2021). IPCC climatic predictions also point to an increase in the intensity and frequency of hot extremes and a decrease in the intensity and frequency of cold extremes for both NWS and nearby SCA (IPCC, 2021). For eastern Panama, Kusunoki et al. (2019) predict an increase between 1 to 2 mm per day in precipitation during the wet season, May to November, for the period 2079–2099 compared to a reference period 1983–2003. Their simulations, based on the atmospheric global model MRI-AGCM3.2, suggest that uncertainties for future precipitation depend upon cumulus convection schemes rather than sea surface temperature distributions. Hidalgo et al. (2017) also suggest wetter conditions in the south-eastern parts of Panama, comparing the period 2029–2049 with 1979–1999, due to a future southward displacement of the Inter-Tropical Convergence Zone. In the face of such uncertain future, as a core activity, Bacurú Drõa has established a permanent forest plot of 10 hectares in 2022 using the methodology of ForestGEO (2022b). By allowing the long-term monitoring of the forest, this plot will enable the Emberá and the scientists to document how these intact primary forests are responding to the unpredictable changing climate.
Indigenous forest custody as a climate change mitigation tool
At the end of this research project, co-author AO expressed frustration that despite the Emberá having protected the Darién Forest and their invaluable ecosystems for centuries, they are receiving no financial compensation to do so. In Balsas, the situation is exacerbated by the fact that they strongly feel that they have not received any benefits from the ~US$10 million Debt-for-Nature-Swap signed by the US and Panama in 2004 to protect the Darién National Park (Natura Panamá, 2021). As a consequence of the paucity of functional international climate policy and incentives to support primary forest conservation and the high profitability of deforestation everywhere in the world, old forests are disappearing at a fast pace (Mackey et al., 2020; Putz, 2020). Besides reporting on the SSP future scenarios and Reasons for Concerns for both ecosystems and human health, the IPCC suggests adopting Climate Resilient Development pathway (IPCC, 2022). In such pathways, adaptation and mitigation actions are taking place at the same time to conserve and restore ecosystems while ensuring decent livelihood. In view of our results, we thus propose three policy options that could help advance toward Climate Resilient Development.
In view of the global disappearance of primary forests, it is crucial to revisit the Good Practice Guidance for Land Use Land Use Change and Forestry (LULUCF) that were adopted in 2003 (IPCC, 2003). These guidelines establish that unmanaged forest land that remains forest land should be excluded from national greenhouse-gas inventories. As a result, old-growth forests become largely invisible to climate policy. Exclusion of forest-land-remaining-forest-land diminishes the perceived importance of forest conservation (Vicuña Miñano et al., 2018). Yet, networks of permanent plots that are ecologically representative allow estimating C fluxes of conserved forests (Phillips et al., 2017). Estimating such fluxes would allow including intact forest C gains in the reporting framework of the UNFCC (Vicuña Miñano et al., 2018; Pirker et al., 2019). Recognition of the role of standing forest in the emissions balance of countries could in turn facilitate their consideration in climate policy.
The core principles of C accounting also demand revisions to facilitate inclusion of forest conservation initiatives at both national and jurisdictional levels. Since the Kyoto Protocol negotiation, land-use related climate actions, such as REDD+, are expected to ensure additionality (new quantified emissions reductions achieved), permanence (emissions reductions not displaced in the future) and leakage (emissions reduction not displaced geographically) (Roopsind et al., 2019). An analysis of the effectiveness of California's Forest Offset Protocol points out to the difficulties of implementing additionality and permanence (Ruseva et al., 2017). While it is easy to prove additionality in the case of a country or a jurisdiction with high rates of deforestation, baseline setting for areas of high forest cover is challenging (Pirker et al., 2019; Roopsind et al., 2019). Recently, an increasing body of literature has used matching techniques to demonstrate the effectiveness of Indigenous territories in protecting forest cover or carbon stocks (Vergara-Asenjo and Potvin, 2014; Blackman and Veit, 2018; Alejo et al., 2021). In these studies, matching was used to compare forest cover or forest cover losses inside and outside Indigenous territories and protected areas. This offers a promising avenue for building credible baselines for forest conservation initiatives thus facilitating their consideration as C offset projects.
Finally, recognizing indigenous land rights is as an essential element of forest conservation strategies (Guèze et al., 2015; Watson et al., 2018). In its 2021 report, the Indigenous Community Conserved Area Consortium recommended the development of “human rights-based financing as a key lever for equitable and effective implementation of global commitments, including on biodiversity, climate and sustainable development” (ICCA Consortium: Worldwide, 2021). Recent pledges at UNFCCC's COP 26, totaling US$1.7 billion to support Indigenous and local communities' tenure rights and security (Sutherland, 2021), could allow advancing in such direction. Novel mechanisms inspired by ecological fiscal transfer (Busch et al., 2021) could possibly be used to support Indigenous forest conservation initiatives. Ecological fiscal transfer indeed compensates jurisdictions within a country for the loss in opportunity costs due to conservation through transfer payment.
Thirty years after the negotiations of the Kyoto Protocol that de facto excluded standing forests from the climate mitigation toolbox and 20 years since the adoption of the LULUCF Good Practice Guidance, time has come to guarantee a sustainable stream of income directly to jurisdictions, such as Indigenous territories, in exchange for their continued commitment to protecting the forests as they have done in the past, according to traditional norms.
Data availability statement
Because this research is a collaboration with Indigenous peoples, any request to obtain raw data and precise locations will first require permission of the Traditional Authorities. If such permission is granted, then data may be made available by the authors.
Ethics statement
The study was reviewed and approved by McGill Research Ethics Board. In addition, we followed the guidelines of the protocol for research in Panama's indigenous communities https://www.mcgill.ca/pfss/files/pfss/protocol.pdf. Because participants were at times illiterate, informed consent to participate in this study was provided either orally or by writing.
Author contributions
CP and MK developed the idea of this study. CP, MK, HB, KR, CM, JV, AO, GL, ID, FG, MD, DP, JP, MO, FS, and TS collected the field data. MK, CP, MG, AK, CM, and GO analyzed the tree and satellite data. DP performed the phytolith analysis. MK, CP, MG, and CM performed the statistical analysis. MK, CP, and DP wrote the first draft of the manuscript and all other co-authors contributed to revising and improving the submitted version. All authors contributed to the article and approved the submitted version.
Funding
The project was funded by an NSERC Discovery Grant (Canada) and a Canada Research Chair to CP as well as a Collaboration International Grant from SENACYT (Panama) to HB.
Acknowledgments
This work would not have been possible without the constant and invaluable help of Lady Mancilla (STRI) and Lupita Omi who herself was supported by the women of Associacion de Mujeres Artesanas y Reforestadora de Ipeti-Emberá (AMARIE). We thank Irene Holst and Graciela Quihana of the STRI Archaeobotanical Laboratory for their technical assistance with the phytolith analysis and the STRI Soil Laboratory for the soil analysis. This manuscript benefited from comments from Camilo Alejo-Monroy, Carmen Umana-Kinitzki, Beth King and Javier Mateo-Vega, we are grateful for their suggestions. We thank Brian Leung and Shiram Varadarajan for sharing with us PRISM's precipitation information for Darién and PlanetLabs for providing the Planet satellite imagery and technical support. We thank RIEGL Laser Measurement Systems GmbH for providing additional batteries for the field and technical support. We thank the SENAFRONT for their service in providing transport logistics and safety support in the field. We also thank the technical assistance of Inga Frehse and Norman Döring at Technische Universität Dresden.
Conflict of interest
The authors declare that the research was conducted in the absence of any commercial or financial relationships that could be construed as a potential conflict of interest.
Publisher's note
All claims expressed in this article are solely those of the authors and do not necessarily represent those of their affiliated organizations, or those of the publisher, the editors and the reviewers. Any product that may be evaluated in this article, or claim that may be made by its manufacturer, is not guaranteed or endorsed by the publisher.
Supplementary material
The Supplementary Material for this article can be found online at: https://www.frontiersin.org/articles/10.3389/fclim.2022.1047832/full#supplementary-material
References
Alcoba, N. (2022). ‘We didn't have choice': Thousands Risk Lives to Cross Darien Gap. Aljazeera. Available online at: https://www.aljazeera.com/news/2022/6/9/we-didnt-have-choice-thousands-risk-lives-to-cross-darien-gap (accessed July 11, 2022).
Alejo, C., Meyer, C., Walker, W. S., Gorelik, S. R., Josse, C., Aragon-Osejo, J. L., et al. (2021). Are indigenous territories effective natural climate solutions? A neotropical analysis using matching methods and geographic discontinuity designs. PLOS ONE 16, e0245110. doi: 10.1371/journal.pone.0245110
Ali, A., and Wang, L.-Q. (2021). Big-sized trees and forest functioning: current knowledge and future perspectives. Ecol. Indic. 127, 107760. doi: 10.1016/j.ecolind.2021.107760
Asner, G. P., Mascaro, J., Anderson, C., Knapp, D. E., Martin, R. E., Kennedy-Bowdoin, T., et al. (2013). High-fidelity national carbon mapping for resource management and REDD+. Carbon Balance Manag. 8, 7. doi: 10.1186/1750-0680-8-7
Banrepcultural (2022). Urabá y Chocó. Encicl. Banrepcultural. Available online at: https://enciclopedia.banrepcultural.org/index.php?title=Urab%C3%A1_y_Choc%C3%B3 (accessed July 11, 2022).
Bastin, J., Rutishauser, E., Kellner, J. R., Saatchi, S., Pélissier, R., Hérault, B., et al. (2018). Pan-tropical prediction of forest structure from the largest trees. Glob. Ecol. Biogeogr. 27, 1366–1383. doi: 10.1111/geb.12803
Bivand, R., Keitt, T., and Rowlingson, B. (2021). rgdal: Bindings for the “Geospatial” Data Abstraction Library. R Package Version 1.5-28. Available online at: https://CRAN.R-project.org/package=rgdal (accessed November 01, 2022).
Bivand, R., and Rundel, C. (2021). rgeos: Interface to Geometry Engine—Open Source (‘GEOS'). R package version 0.5-9. Available online at: https://CRAN.R-project.org/package=rgeos (accessed November 01, 2022).
Blackman, A., and Veit, P. (2018). Titled Amazon indigenous communities cut forest carbon emissions. Ecol. Econ. 153, 56–67. doi: 10.1016/j.ecolecon.2018.06.016
Blair, L. (2022). Risking it all: migrants brave Darién Gap in pursuit of the American dream. The Guardian. Available online at: https://www.theguardian.com/global-development/2022/apr/28/risking-it-all-migrants-brave-darien-gap-in-pursuit-of-the-american-dream (accessed July 11, 2022).
Boettinger, J. L., Ramsey, R. D., Bodily, J. M., Cole, N. J., Kienast-Brown, S., Nield, S. J., et al. (2008). “Landsat spectral data for digital soil mapping,” in Digital Soil Mapping with Limited Data, eds A. E. Hartemink, A. McBratney, and M. de L. Mendonça-Santos (Dordrecht: Springer Netherlands), 193–202. doi: 10.1007/978-1-4020-8592-5_16
Boulton, C. A., Lenton, T. M., and Boers, N. (2022). Pronounced loss of Amazon rainforest resilience since the early 2000s. Nat. Clim. Change 12, 271–278. doi: 10.1038/s41558-022-01287-8
Bryant, D. G., Nielsen, D., and Tangley, L. (1997). The Last Frontier Forests: Ecosystems and Economies on the Edge: What is the Status of the World's Remaining Large, Natural Forest Ecosystems? Washington, DC: World Resources Institute, Forest Frontiers Initiative.
Busch, J., Ring, I., Akullo, M., Amarjargal, O., Borie, M., Cassola, R. S., et al. (2021). A global review of ecological fiscal transfers. Nat. Sustain. 4, 756–765. doi: 10.1038/s41893-021-00728-0
Bush, M. B., McMichael, C. H., Piperno, D. R., Silman, M. R., Barlow, J., Peres, C. A., et al. (2015). Anthropogenic influence on Amazonian forests in pre-history: an ecological perspective. J. Biogeogr. 42, 2277–2288. doi: 10.1111/jbi.12638
Bush, M. B., Piperno, D. R., Colinvaux, P. A., De Oliveira, P. E., Krissek, L. A., Miller, M. C., et al. (1992). A 14300-yr paleoecological profile of a lowland tropical lake in Panama. Ecol. Monogr. 62, 251–275. doi: 10.2307/2937095
Castellanos, E., Lemos, M. F., Astigarraga, N., Chacón, N., Cuvi, N., Huggel, C., et al. (2022). “Central and South America,” in Climate Change 2022: Impacts, Adaptation, and Vulnerability. Contribution of Working Group II to the Sixth Assessment Report of the Intergovernmental Panel on Climate Change, eds H.-O. Pörtner, D. C. Roberts, M. Tignor, E. S. Poloczanska, K. Mintenbeck (Central and South America), 1689–1816. doi: 10.1017/9781009325844.014
Clark, D. B., Ferraz, A., Clark, D. A., Kellner, J. R., Letcher, S. G., and Saatchi, S. (2019). Diversity, distribution and dynamics of large trees across an old-growth lowland tropical rain forest landscape. PLOS ONE 14, e0224896. doi: 10.1371/journal.pone.0224896
Colectivo Darién (2021). Trafficking as settler colonialism in eastern Panama: linking the Americas via illicit commerce, clientelism, and land cover change. World Dev. 145, 105490. doi: 10.1016/j.worlddev.2021.105490
Condit, R., Aguilar, S., and Pérez, R. (2020). Trees of Panama: a complete checklist with every geographic range. For. Ecosyst. 7, 42. doi: 10.1186/s40663-020-00246-z
Coomes, O. T., Kalacska, M., Takasaki, Y., Abizaid, C., and Grupp, T. (2022). Smallholder agriculture results in stable forest cover in riverine Amazonia. Environ. Res. Lett. 17, 014024. doi: 10.1088/1748-9326/ac417c
Drost, N. (2020). What migrants face as they journey through the deadly Darien Gap. PBS News Hour. Available online at: https://www.pbs.org/newshour/show/what-migrants-face-as-they-journey-through-the-deadly-darien-gap (accessed November 01, 2022).
ESA Copernicus (2022). Copernicus Open Access Hub. Available online at: https://scihub.copernicus.eu/dhus/#/home (accessed October 30, 2022).
Esquivel-Muelbert, A., Baker, T. R., Dexter, K. G., Lewis, S. L., Brienen, R. J. W., Feldpausch, T. R., et al. (2019). Compositional response of Amazon forests to climate change. Glob. Change Biol. 25, 39–56. doi: 10.1111/gcb.14413
ETASA (2022). Lista de Estaciones Meteorológicas. Available online at: https://www.hidromet.com.pa/es/estaciones-meteorologicas?f_numero=andf_nombre=andf_cuenca=noopcionandf_institucion=noopcionandf_provincia=DARIEN (accessed November 8, 2022).
Fearnside, P. M. (2001). Saving tropical forests as a global warming countermeasure: an issue that divides the environmental movement. Ecol. Econ. 39, 167–184. doi: 10.1016/S0921-8009(01)00225-7
ForestGEO (2022a). ForestGEO Data Portal. For. Data Portal. Available online at: http://ctfs.si.edu/datarequest/index.php/main
ForestGEO (2022b). ForestGEO Protocols. Available online at: https://www.forestgeo.si.edu/protocols (accessed November 1, 2022).
Forrester, D. I. (2021). Does individual-tree biomass growth increase continuously with tree size? For. Ecol. Manag. 481, 118717. doi: 10.1016/j.foreco.2020.118717
Frechette, J. E. M., Ginsburg, C., and Walker, W. (2018). A Global Baseline of Carbon Storage in Collective Lands. Indigenous and Local Community Contributions to Climate Change Mitigation. Washington, DC: Rights and Resources Initiative.
Garnett, S. T., Burgess, N. D., Fa, J. E., Fernández-Llamazares, Á., Molnár, Z., Robinson, C. J., et al. (2018). A spatial overview of the global importance of Indigenous lands for conservation. Nat. Sustain. 1, 369–374. doi: 10.1038/s41893-018-0100-6
Gaviria, C. M. H. (2018). Espacio salvaje y colonialismo global en el Darién, Colombia, 1826-1924. Rev. Direito Cid. 10, 34211. doi: 10.12957/rdc.2018.34211
Ghazoul, J., Burivalova, Z., Garcia-Ulloa, J., and King, L. A. (2015). Conceptualizing forest degradation. Trends Ecol. Evol. 30, 622–632. doi: 10.1016/j.tree.2015.08.001
Gibson, L., Lee, T. M., Koh, L. P., Brook, B. W., Gardner, T. A., Barlow, J., et al. (2011). Primary forests are irreplaceable for sustaining tropical biodiversity. Nature 478, 378–381. doi: 10.1038/nature10425
Gorenflo, L. J., Romaine, S., Mittermeier, R. A., and Walker-Painemilla, K. (2012). Co-occurrence of linguistic and biological diversity in biodiversity hotspots and high biodiversity wilderness areas. Proc. Natl. Acad. Sci. USA 109, 8032–8037. doi: 10.1073/pnas.1117511109
Gorgens, E. B., Nunes, M. H., Jackson, T., Coomes, D., Keller, M., Reis, C. R., et al. (2021). Resource availability and disturbance shape maximum tree height across the Amazon. Glob. Change Biol. 27, 177–189. doi: 10.1111/gcb.15423
Gray, C., and Bilsborrow, R. (2020). Stability and change within indigenous land use in the Ecuadorian Amazon. Glob. Environ. Change 63, 102116. doi: 10.1016/j.gloenvcha.2020.102116
Griscom, B. W., Adams, J., Ellis, P. W., Houghton, R. A., Lomax, G., Miteva, D. A., et al. (2017). Natural climate solutions. Proc. Natl. Acad. Sci. USA 114, 11645–11650. doi: 10.1073/pnas.1710465114
Guèze, M., Luz, A. C., Paneque-Gálvez, J., Macía, M. J., Orta-Martínez, M., Pino, J., et al. (2015). Shifts in indigenous culture relate to forest tree diversity: a case study from the Tsimane, Bolivian Amazon. Biol. Conserv. 186, 251–259. doi: 10.1016/j.biocon.2015.03.026
Hansen, M. C., Potapov, P. V., Moore, R., Hancher, M., Turubanova, S. A., Tyukavina, A., et al. (2013). High-resolution global maps of 21st-century forest cover change. Science 342, 850–853. doi: 10.1126/science.1244693
Harris, D. J., Ndolo Ebika, S. T., Sanz, C. M., Madingou, M. P. N., and Morgan, D. B. (2021). Large trees in tropical rain forests require big plots. Plants People Planet 3, 282–294. doi: 10.1002/ppp3.10194
Harvey, C. A., Saborio-Rodríguez, M., Martinez-Rodríguez, M. R., Viguera, B., Chain-Guadarrama, A., Vignola, R., et al. (2018). Climate change impacts and adaptation among smallholder farmers in Central America. Agric. Food Secur. 7, 57. doi: 10.1186/s40066-018-0209-x
Henderson, A., Galeano-Garces, G., and Bernal, R. (1995). Field Guide to the Palms of the Americas. Princeton: Princeton University Press.
Herlihy, P. H. (1985). Settlement and Subsistence Change Among the Chocó Indians of the Darién Province, Eastern Panama: An Overview. Hammond, IN: Southeastern Louisiana University.
Herlihy, P. H. (1986). A Cultural Geography of the Emberá and Wounan (Choco) Indians of Darién, Panama, With Emphasis on Recent Village Formation and Economic Diversification. LSU Historical Dissertations and Theses, 4299. Louisiana State University (LSU).
Hidalgo, H. G., Alfaro, E. J., and Quesada-Montano, B. (2017). Observed (1970–1999) climate variability in Central America using a high-resolution meteorological dataset with implication to climate change studies. Clim. Change 141, 13–28. doi: 10.1007/s10584-016-1786-y
Hijmans, R. J. (2022). raster: Geographic Data Analysis and Modeling. R package version 3.5-15. Available online at: https://CRAN.R-project.org/package=raster (accessed November 01, 2022).
ICCA Consortium: Worldwide (2021). Territories of Life 2021 Report. ICCA Consortium. Available online at: https://report.territoriesoflife.org/ (accessed November 01, 2022).
IDIAP (2010). Mapa de fertilidad basado en análisis de muestras de suelos: Texturas. Panama: Instituto de Investigación Agropecuaria de Panamá, Ministerio de Desarrollo Agropecuario, República de Panamá.
IPCC (2003). Good Practice Guidance for Land Use, Land-Use Change and Forestry, ed. J. Penman Hayama. Kanagawa: IPCC.
IPCC (2021). “Climate change 2021: the physical science basis,” in Contribution of Working Group I to the Sixth Assessment Report of the Intergovernmental Panel on Climate Change, eds V. Masson-Delmotte, P. Zhai, A. Pirani, S. L. Connors, C. Péan, S. Berger, et al. (Cambridge and New York: Cambridge University Press), 2391. doi: 10.1017/9781009157896
IPCC (2022). “IPCC, 2022: Climate Change 2022: Impacts, Adaptation and Vulnerability,” in Contribution of Working Group II to the Sixth Assessment Report of the Intergovernmental Panel on Climate Change, eds H.-O. Pörtner, D. C. Roberts, M. Tignor, E. S. Poloczanska, K. Mintenbeck, A. Alegría, et al. (Cambridge and New York: Cambridge University Press), 3056. doi: 10.1017/9781009325844
Jacobs, D. F., Oliet, J. A., Aronson, J., Bolte, A., Bullock, J. M., Donoso, P. J., et al. (2015). Restoring forests: what constitutes success in the twenty-first century? New For. 46, 601–614. doi: 10.1007/s11056-015-9513-5
Johnson, D. V., and IUCN/SSC Palm Specialist Group, (eds) (1996). Palms: Their Conservation and Sustained Utilization: Status Survey and Conservation Action Plan. Gland, Switzerland: IUCN.
Jucker, T., Fischer, F. J., Chave, J., Coomes, D. A., Caspersen, J., Ali, A., et al. (2022). Tallo: a global tree allometry and crown architecture database. Glob. Change Biol. 2022, 16302. doi: 10.1111/gcb.16302
Kim, D.-H., Sexton, J. O., Noojipady, P., Huang, C., Anand, A., Channan, S., et al. (2014). Global, Landsat-based forest-cover change from 1990 to 2000. Remote Sens. Environ. 155, 178–193. doi: 10.1016/j.rse.2014.08.017
King, D. A., Davies, S. J., Tan, S., and Md. Noor, N. S. (2006). The role of wood density and stem support costs in the growth and mortality of tropical trees: tree demography and stem support costs. J. Ecol. 94, 670–680. doi: 10.1111/j.1365-2745.2006.01112.x
Kolanowska, M. (2015). Determination of potential glacial refugia and possible migration routes of Campylocentrum (Vandeae, Orchidaceae) species through the Darién Gap. Acta Soc. Bot. Pol. 84, 97–102. doi: 10.5586/asbp.2014.030
Kothari A. Corrigan C. Jonas H. Neumann A. Shrumm H. Secretariat of the Convention on Biological Diversity (2014). Recognising and Supporting Territories and Areas Conserved by Indigenous Peoples and Local Communities: Global Overview and National Case Studies. Available online at: http://www.deslibris.ca/ID/242837 (accessed August 17, 2022).
Kusunoki, S., Nakaegawa, T., Pinzón, R., Sanchez-Galan, J. E., and Fábrega, J. R. (2019). Future precipitation changes over Panama projected with the atmospheric global model MRI-AGCM3.2. Clim. Dyn. 53, 5019–5034. doi: 10.1007/s00382-019-04842-w
LAStools. (2020). LAStools, version 201207 (academic), rapidlasso GmbH, Germany. Available online at: https://rapidlasso.com/lastools/
Levis, C., Flores, B. M., Moreira, P. A., Luize, B. G., Alves, R. P., Franco-Moraes, J., et al. (2018). How people domesticated Amazonian forests. Front. Ecol. Evol. 5, 171. doi: 10.3389/fevo.2017.00171
Lewis, S. L., Wheeler, C. E., Mitchard, E. T. A., and Koch, A. (2019). Restoring natural forests is the best way to remove atmospheric carbon. Nature 568, 25–28. doi: 10.1038/d41586-019-01026-8
Lindenmayer, D. B., Blanchard, W., McBurney, L., Blair, D., Banks, S., Likens, G. E., et al. (2012). Interacting factors driving a major loss of large trees with cavities in a forest ecosystem. PLoS ONE 7, e41864. doi: 10.1371/journal.pone.0041864
Lutz, J. A., Furniss, T. J., Johnson, D. J., Davies, S. J., Allen, D., Alonso, A., et al. (2018). Global importance of large-diameter trees. Glob. Ecol. Biogeogr. 27, 849–864. doi: 10.1111/geb.12747
Mackey, B., Berry, S., Hugh, S., Ferrier, S., Harwood, T. D., and Williams, K. J. (2012). Ecosystem greenspots: identifying potential drought, fire, and climate-change micro-refuges. Ecol. Appl. 22, 1852–1864. doi: 10.1890/11-1479.1
Mackey, B., DellaSala, D. A., Kormos, C., Lindenmayer, D., Kumpel, N., Zimmerman, B., et al. (2015). Policy options for the World's primary forests in multilateral environmental agreements. Conserv. Lett. 8, 139–147. doi: 10.1111/conl.12120
Mackey, B., Kormos, C. F., Keith, H., Moomaw, W. R., Houghton, R. A., Mittermeier, R. A., et al. (2020). Understanding the importance of primary tropical forest protection as a mitigation strategy. Mitig. Adapt. Strateg. Glob. Change 25, 763–787. doi: 10.1007/s11027-019-09891-4
Mateo-Vega, J., Arroyo-Mora, J. P., and Potvin, C. (2019). Tree aboveground biomass and species richness of the mature tropical forests of Darien, Panama, and their role in global climate change mitigation and biodiversity conservation. Conserv. Sci. Pract. 1, e42. doi: 10.1111/csp2.42
Mateo-Vega, J., Potvin, C., Monteza, J., Bacorizo, J., Barrigón, J., Barrigón, R., et al. (2017). Full and effective participation of indigenous peoples in forest monitoring for reducing emissions from deforestation and forest degradation (REDD+): trial in Panama's Darién. Ecosphere 8, e01635. doi: 10.1002/ecs2.1635
McMichael, C. H., Piperno, D. R., Bush, M. B., Silman, M. R., Zimmerman, A. R., Raczka, M. F., et al. (2012). Sparse pre-columbian human habitation in Western Amazonia. Science 336, 1429–1431. doi: 10.1126/science.1219982
Meyer, V., Saatchi, S., Ferraz, A., Xu, L., Duque, A., García, M., et al. (2019). Forest degradation and biomass loss along the Chocó region of Colombia. Carbon Balance Manag. 14, 2. doi: 10.1186/s13021-019-0117-9
Ministerio de Ambiente (2019). Resolución N° DM-0612-2019. República de Panamá, Goberierno Nacional. Available online at: https://www.gacetaoficial.gob.pa/pdfTemp/28912_A/GacetaNo_28912a_20191202.pdf
Mitchard, E. T. A. (2018). The tropical forest carbon cycle and climate change. Nature 559, 527–534. doi: 10.1038/s41586-018-0300-2
Mollicone, D., Achard, F., Federici, S., Eva, H. D., Grassi, G., Belward, A., et al. (2007). An incentive mechanism for reducing emissions from conversion of intact and non-intact forests. Clim. Change 83, 477–493. doi: 10.1007/s10584-006-9231-2
Morcote-Ríos, G., Bernal, R., and Raz, L. (2016). Phytoliths as a tool for archaeobotanical, palaeobotanical and palaeoecological studies in Amazonian palms. Bot. J. Linn. Soc. 182, 348–360. doi: 10.1111/boj.12438
Natura Panamá (2021). Darien Debt for Nature Swap Objectives. Available online at: https://naturapanama.org/darien-fund/?lang=en (accessed November 1, 2022).
Pebesma, E. (2018). Simple features for R: standardized support for spatial vector data. R J. 10, 439. doi: 10.32614/RJ-2018-009
Pereira Carneiro, C., Reolon, C. A., and Portela, J. P. (2019). A rodovia Pan-Americana e o Tampão de Darién: integração continental e áreas protegidas em zona de fronteira. Rev. Transp. Territ. 21, 28–43. doi: 10.34096/rtt.i21.7145
Phillips O. L. Brienen R. J. W. the RAINFOR collaboration (2017). Carbon uptake by mature Amazon forests has mitigated Amazon nations' carbon emissions. Carbon Balance Manag. 12, 1. doi: 10.1186/s13021-016-0069-2
Piperno, D. R. (1994). Phytolith and charcoal evidence for prehistoric slash-and-burn agriculture in the Darien rain forest of Panama. Holocene 4, 321–325. doi: 10.1177/095968369400400312
Piperno, D. R. (2006). Phytoliths: A Comprehensive Guide for Archaeologists and Paleoecologists. Lanham, MD: AltaMira Press.
Piperno, D. R., McMichael, C., and Bush, M. B. (2015). Amazonia and the Anthropocene: what was the spatial extent and intensity of human landscape modification in the Amazon Basin at the end of prehistory? Holocene 25, 1588–1597. doi: 10.1177/0959683615588374
Piperno, D. R., McMichael, C. H., Pitman, N. C. A., Andino, J. E. G., Ríos Paredes, M., Heijink, B. M., et al. (2021). A 5,000-year vegetation and fire history for tierra firme forests in the Medio Putumayo-Algodón watersheds, northeastern Peru. Proc. Natl. Acad. Sci. USA 118, e2022213118. doi: 10.1073/pnas.2022213118
Pirker, J., Mosnier, A., Nana, T., Dees, M., Momo, A., Muys, B., et al. (2019). Determining a carbon reference level for a high-forest-low-deforestation country. Forests 10, 1095. doi: 10.3390/f10121095
Planet Labs PBC (2022). Planet Explorer. Available online at: https://developers.planet.com/docs/apps/explorer/ (accessed October 31, 2022).
Posey, D. (1985). Indigenous management of tropical forest ecosystems: the case of the Kayapo indians of the Brazilian Amazon. Agrofor. Syst. 3, 139–158.
Potapov, P., Hansen, M. C., Laestadius, L., Turubanova, S., Yaroshenko, A., Thies, C., et al. (2017). The last frontiers of wilderness: tracking loss of intact forest landscapes from 2000 to 2013. Sci. Adv. 3, e1600821. doi: 10.1126/sciadv.1600821
Potvin, C., Barrios, H., and Dan, M. (2020). Establishing Historical Presence of Emberá Communities in Río Balsas. Montreal, QC: Department of Biology, McGill University.
Putz, F. E. (2020). Science-to-conservation disconnections in Borneo and British Columbia. For. Chron. 96, 20–26. doi: 10.5558/tfc2020-004
Roopsind, A., Sohngen, B., and Brandt, J. (2019). Evidence that a national REDD+ program reduces tree cover loss and carbon emissions in a high forest cover, low deforestation country. Proc. Natl. Acad. Sci. USA 116, 24492–24499. doi: 10.1073/pnas.1904027116
Rozendaal, D. M. A., Bongers, F., Aide, T. M., Alvarez-Dávila, E., Ascarrunz, N., Balvanera, P., et al. (2019). Biodiversity recovery of Neotropical secondary forests. Sci. Adv. 5, eaau3114. doi: 10.1126/sciadv.aau3114
Rüger, N., Condit, R., Dent, D. H., DeWalt, S. J., Hubbell, S. P., Lichstein, J. W., et al. (2020). Demographic trade-offs predict tropical forest dynamics. Science 368, 165–168. doi: 10.1126/science.aaz4797
Runk, J. V. (2015). Creating Wild Darién: centuries of Darién's imaginative geography and its lasting effects. J. Lat. Am. Geogr. 14, 127–156. doi: 10.1353/lag.2015.0032
Ruseva, T., Marland, E., Szymanski, C., Hoyle, J., Marland, G., and Kowalczyk, T. (2017). Additionality and permanence standards in California's forest offset protocol: a review of project and program level implications. J. Environ. Manage. 198, 277–288. doi: 10.1016/j.jenvman.2017.04.082
Saleska, S. R., Didan, K., Huete, A. R., and da Rocha, H. R. (2007). Amazon forests green-up during 2005 drought. Science 318, 612–612. doi: 10.1126/science.1146663
Sangha, K. K. (2020). Global importance of indigenous and local communities' managed lands: building a case for stewardship schemes. Sustainability 12, 7839. doi: 10.3390/su12197839
Santos-Granero, F. (1998). Writing history into the landscape: space, myth, and ritual in contemporary Amazonia. Am. Ethnol. 25, 128–148.
Santos-Granero, F. (2004). In Darkness and Secrecy—The Anthropology of Assault Sorcery and Witchcraft in Amazonia. eds. N. L. Whitehead and R. Wright (Durham and London: Duke University Press).
Schneider, E., Cámara-Leret, R., Barfod, A., and Weckerle, C. S. (2017). Palm use by two chachi communities in ecuador: a 30-year reappraisal. Econ. Bot. 71, 342–360. doi: 10.1007/s12231-017-9397-8
Sheil, D., Eastaugh, C. S., Vlam, M., Zuidema, P. A., Groenendijk, P., Sleen, P., et al. (2017). Does biomass growth increase in the largest trees? Flaws, fallacies and alternative analyses. Funct. Ecol. 31, 568–581. doi: 10.1111/1365-2435.12775
Sietsma, A. J., Ford, J. D., Callaghan, M. W., and Minx, J. C. (2021). Progress in climate change adaptation research. Environ. Res. Lett. 16, 054038. doi: 10.1088/1748-9326/abf7f3
Sist, P., Mazzei, L., Blanc, L., and Rutishauser, E. (2014). Large trees as key elements of carbon storage and dynamics after selective logging in the Eastern Amazon. For. Ecol. Manag. 318, 103–109. doi: 10.1016/j.foreco.2014.01.005
Slik, J. W. F., Paoli, G., McGuire, K., Amaral, I., Barroso, J., Bastian, M., et al. (2013). Large trees drive forest aboveground biomass variation in moist lowland forests across the tropics: Large trees and tropical forest biomass. Glob. Ecol. Biogeogr. 22, 1261–1271. doi: 10.1111/geb.12092
Sobrevila, C. (2008). The Role of Indigenous Peoples in Biodiversity Conservation—The Natural but Often Forgotten Partners. Washington DC: The World Bank.
Stephenson, N. L., Das, A. J., Condit, R., Russo, S. E., Baker, P. J., Beckman, N. G., et al. (2014). Rate of tree carbon accumulation increases continuously with tree size. Nature 507, 90–93. doi: 10.1038/nature12914
Stiglitz, J. E. (2005). Cleaning Up Economic Growth. Project Syndicate Print Commentary. Project Syndicate. Available online at: https://www.project-syndicate.org/commentary/cleaning-up-economic-growth (accessed November 1, 2022).
Streck, C., and Scholz, S. M. (2006). The role of forests in global climate change: whence we come and where we go. Int. Aff. 82, 861–879. doi: 10.1111/j.1468-2346.2006.00575.x
Suárez Pinzón, I. (2011). Darien province and Panamá isthmus: three centuries in the heart of disputes for capitalist expansion. Anu. Hist. Reg. Las Front. 16, 17–50.
Suman, D. (2007). Globalization and the Pan-American highway: converns for the panama-columbia border region of Darién-Chocó and its peoples. U Miami Inter-Am. Rev. 38. Available online at: http://repository.law.miami.edu/umialr/vol38/iss3/3
Sutherland, L. (2021). $1.7 Billion Pledged in Support of Indigenous and Local Communities' Land Tenure. Available online at: https://news.mongabay.com/2021/11/1-7-billion-pledged-in-support-of-indigenous-and-local-communities-land-tenure/ (accessed November 2, 2021).
Tambosi, L. R., Martensen, A. C., Ribeiro, M. C., and Metzger, J. P. (2014). A framework to optimize biodiversity restoration efforts based on habitat amount and landscape connectivity: optimizing restoration based on landscape resilience. Restor. Ecol. 22, 169–177. doi: 10.1111/rec.12049
UNESCO World Heritage (1981). Darien National Park. Available online at: https://whc.unesco.org/en/list/159/ (accessed November 1, 2022).
USGS (2022). Landsat Known Issues. Available online at: https://www.usgs.gov/landsat-missions/landsat-known-issues (accessed November 1, 2022).
Varadarajan, S., Fábrega, J., and Leung, B. (2022). Precipitation interpolation, autocorrelation, and predicting spatiotemporal variation in runoff in data sparse regions: application to Panama. J. Hydrol. Reg. Stud. 44, 101252. doi: 10.1016/j.ejrh.2022.101252
Vardeman, E., and Runk, J. V. (2020). Panama's illegal rosewood logging boom from Dalbergia retusa. Glob. Ecol. Conserv. 23, e01098. doi: 10.1016/j.gecco.2020.e01098
Vergara-Asenjo, G., and Potvin, C. (2014). Forest protection and tenure status: the key role of indigenous peoples and protected areas in Panama. Glob. Environ. Change 28, 205–215. doi: 10.1016/j.gloenvcha.2014.07.002
Vicuña Miñano, E., Baker, T. R., Banda, R. K, Honorio Coronado, E., Monteagudo, A., Phillips, O. L., et al. (2018). El sumidero de carbono en los bosques primarios Amazónicos es una oportunidad para lograr la sostenibilidad de su conservación. Folia Amaz. 27, 101–109. doi: 10.24841/fa.v27i1.456
Wang, L.-Q., and Ali, A. (2021). Climate regulates the functional traits—aboveground biomass relationships at a community-level in forests: a global meta-analysis. Sci. Total Environ. 761, 143238. doi: 10.1016/j.scitotenv.2020.143238
Watson, J. E. M., Evans, T., Venter, O., Williams, B., Tulloch, A., Stewart, C., et al. (2018). The exceptional value of intact forest ecosystems. Nat. Ecol. Evol. 2, 599–610. doi: 10.1038/s41559-018-0490-x
Wessel, P., Smith, W. H. F., Scharroo, R., Luis, J., and Wobbe, F. (2013). Generic mapping tools: improved version released. Eos Trans. Am. Geophys. Union 94, 409–410. doi: 10.1002/2013EO450001
WHRC and EDF (2015). Tropical Forest Carbon in Indigenous Territories: A Global Analysis. A report prepared for UNFCCC COP 21. Falmouth, MA and Washington, DC, USA: The Woods Hole Research Center and Environmental Defense Fund, WHRC [Woods Hole Research Center] and EDF [Environmental Defense Fund].
Wickham, H. (2016). ggplot2: Elegant Graphics for Data Analysis. 2nd ed. Cham: Springer International Publishing.
Wilson, A. M., and Jetz, W. (2016). Remotely sensed high-resolution global cloud dynamics for predicting ecosystem and biodiversity distributions. PLOS Biol. 14, e1002415. doi: 10.1371/journal.pbio.1002415
Witteveen, N. H., Hobus, C. E. M., Philip, A., Piperno, D. R., and McMichael, C. N. H. (2022). The variability of Amazonian palm phytoliths. Rev. Palaeobot. Palynol. 300, 104613. doi: 10.1016/j.revpalbo.2022.104613
Keywords: large trees, phytoliths, land use, Indigenous peoples, old-growth tropical forests, terrestrial laser scanning, climate change, carbon finance
Citation: Kunz M, Barrios H, Dan M, Dogirama I, Gennaretti F, Guillemette M, Koller A, Madsen C, Lana G, Ortega A, Ortega M, Paripari J, Piperno D, Reich KF, Simon T, Solis F, Solis P, Valdes J, von Oheimb G and Potvin C (2022) Bacurú Drõa: Indigenous forest custody as an effective climate change mitigation option. A case study from Darién, Panama. Front. Clim. 4:1047832. doi: 10.3389/fclim.2022.1047832
Received: 18 September 2022; Accepted: 18 November 2022;
Published: 07 December 2022.
Edited by:
Nathan S. Debortoli, PlanAdapt Collaborative gUG, GermanyReviewed by:
Rafael Coll Delgado, Universidade Federal Rural do Rio de Janeiro, BrazilDaniel Zacarias, Eduardo Mondlane University, Mozambique
Copyright © 2022 Kunz, Barrios, Dan, Dogirama, Gennaretti, Guillemette, Koller, Madsen, Lana, Ortega, Ortega, Paripari, Piperno, Reich, Simon, Solis, Solis, Valdes, von Oheimb and Potvin. This is an open-access article distributed under the terms of the Creative Commons Attribution License (CC BY). The use, distribution or reproduction in other forums is permitted, provided the original author(s) and the copyright owner(s) are credited and that the original publication in this journal is cited, in accordance with accepted academic practice. No use, distribution or reproduction is permitted which does not comply with these terms.
*Correspondence: Matthias Kunz, matthias.kunz@tu-dresden.de; Catherine Potvin, catherine.potvin@mcgill.ca
†ORCID: Hector Barrios orcid.org/0000-0002-0542-1731