Development, Pathogenesis, and Regeneration of the Intervertebral Disc: Current and Future Insights Spanning Traditional to Omics Methods
- 1Department of Medicine, Vanderbilt University Medical Center, Nashville, TN, United States
- 2Division of Clinical Pharmacology, Vanderbilt University Medical Center, Nashville, TN, United States
- 3Vanderbilt Center for Bone Biology, Vanderbilt University Medical Center, Nashville, TN, United States
The intervertebral disc (IVD) is the fibrocartilaginous joint located between each vertebral body that confers flexibility and weight bearing capabilities to the spine. The IVD plays an important role in absorbing shock and stress applied to the spine, which helps to protect not only the vertebral bones, but also the brain and the rest of the central nervous system. Degeneration of the IVD is correlated with back pain, which can be debilitating and severely affects quality of life. Indeed, back pain results in substantial socioeconomic losses and healthcare costs globally each year, with about 85% of the world population experiencing back pain at some point in their lifetimes. Currently, therapeutic strategies for treating IVD degeneration are limited, and as such, there is great interest in advancing treatments for back pain. Ideally, treatments for back pain would restore native structure and thereby function to the degenerated IVD. However, the complex developmental origin and tissue composition of the IVD along with the avascular nature of the mature disc makes regeneration of the IVD a uniquely challenging task. Investigators across the field of IVD research have been working to elucidate the mechanisms behind the formation of this multifaceted structure, which may identify new therapeutic targets and inform development of novel regenerative strategies. This review summarizes current knowledge base on IVD development, degeneration, and regenerative strategies taken from traditional genetic approaches and omics studies and discusses the future landscape of investigations in IVD research and advancement of clinical therapies.
Introduction
The intervertebral disc (IVD) is a fibrocartilaginous structure that connects the vertebral bodies in the spine. The IVD is composed of three substructures: 1) the proteoglycan-rich central region referred to as the nucleus pulposus (NP), 2) the fibrocartilaginous peripheral region referred to as the annulus fibrosus (AF), and 3) the superior and inferior cartilaginous endplates (CEP) (Humzah and Soames, 1988; Cassidy et al., 1989). The main role of the IVD is to provide structural support to the spinal column by absorbing and transferring the compressive forces that are applied to the spine and to confer mobility to the spine.
The IVD is particularly prone to impaired repair leading to permanent damage. The IVD, which is the largest avascular organ in the body, has low cell density, low nutrient flow, and a low oxygen environment, creating a particularly harsh microenvironment for the resident cells (Diamant et al., 1968; Ishihara and Urban, 1999). Thus, these cells tend to be much less metabolically active, even in healthy states (Bibby et al., 2005). IVD degeneration (IDD) and associated back pain is a major socioeconomic burden, affecting 85% of the population at some point in their lifetime and costing over $100 billion annually in the United States (Katz, 2006; Hoy et al., 2014; Buchbinder et al., 2018). While IDD has been implicated as a major cause of back pain, the direct mechanistic links to pathology have not been fully elucidated. Current treatments for back pain include physical therapy, anti-inflammatory medications, and in severe cases, artificial disc replacement or spinal fusion (Mirza and Deyo, 2007). None of these treatment approaches recapitulate native structure and function of the disc, and the primary focus is on alleviating or managing pain. Thus, there is a need for development of therapies that can restore disc structure and functional mechanics while alleviating the painful symptoms associated with IDD and back pain. As such, there has been much interest in developing regenerative therapies to apply to the IVD.
Regenerative strategies that have garnered much interest in recent years are focused on elucidating the native developmental processes of the IVD to apply early developmental signals for in vivo tissue regeneration by combining tissue engineering using biomimetic scaffolds, targeted drug delivery for endogenous cell activation and differentiation, and exogenous cell implantation (Pirvu et al., 2014; Pirvu et al., 2015; Richardson et al., 2016; Bowles and Setton, 2017; Baumgartner et al., 2021; Du et al., 2021). Improved understanding of intervertebral disc formation may enable reproduction of important developmental signals in the degenerated or injured discs as a regenerative strategy. Elucidating a fuller profile of the specific molecular signals that direct tissue formation and applying these signals to therapeutic cell types like mesenchymal stem cells (MSCs) or induced pluripotent stem cells (iPSCs) could potentially drive these cells towards AF or NP-like phenotypes that can regenerate the damaged tissue. While many laboratory studies have pointed to potential successful application of pluripotent and multipotent therapeutic cell types in regenerative medicine, many important considerations remain under investigation. Fully pluripotent stem cells like embryonic stem cells (ESCs) are theoretically the ideal cell type in terms of regenerative potential, but ethical concerns, limited cell numbers and sources for harvest, and immunologic reactions after implantation hinder application of these cells. On the other hand, MSCs are plentiful and do not carry the same ethical burdens as they can be harvested from multiple tissues even from adult donors and seem to induce minimal or no immunologic response. However, limited proliferative capability and cell senescence remain hurdles for widespread application of MSCs. Importantly, the IVD is a particularly harsh environment for implanted cell survival, differentiation, and highly synthetic processes like tissue formation, and as such, continued studies delving into the multi-faceted aspects of the IVD to inform effective therapeutic development have remained a hotly pursued area of investigation.
To this end, animal models, particularly genetic mouse models, have provided critical insights into genetic and molecular profiles of IVD development. In addition to murine models, larger animals such as rabbit, dog, sheep, and bovine have played an important role as their IVDs are more similar in size and mechanical load to human IVDs, particularly in studies involving biomechanical changes over time and the application of pre-clinical therapies (Alini et al., 2008; Sakai and Andersson, 2015). While challenges in translating findings from animal models to human patients due to differences in important factors such as IVD size, cell turnover, and biomechanical load need to be acknowledged and carefully considered, the usefulness of these experimental models cannot be ignored. Furthermore, the advent of omics technologies like transcriptomics, proteomics, and metabolomics have allowed researchers to make significant progress in understanding global profiles and longitudinal changes in the IVD, both during development and in degeneration. Although human samples are much more difficult to collect, especially in large cohorts, these technical advances have allowed researchers to gain insight into gene and protein expression profiles of the human IVD at different developmental stages as discussed below. With these omics technologies becoming widely available to most research labs, both in terms of cost and accessibility, in-depth analyses of IVD animal models have become commonplace in recent years. This allows for wide-reaching comparative studies between different types of animal models as well as comparisons with findings from human patient samples, all efforts that have been pointing the IVD research community toward better consensus on important topics such as the molecular basis of IVD development, maintenance, and degeneration, and molecular markers that define various cells and components within the IVD. Collectively, the combined growing breadth and depth of available data in all aspects of IVD research from both human patient samples and animal models will inform novel diagnostics and therapeutics that will ultimately address the major clinical need of developing long-term IDD treatments.
This review summarizes the currently known aspects of IVD development, degeneration, and therapeutics taken from traditional and omics studies as well as the future landscape of clinical treatment and regenerative strategies in IVD research.
Intervertebral Disc Composition
The IVD is comprised of three substructures: the nucleus pulposus (NP), annulus fibrosus (AF), and two endplates of hyaline cartilage on the superior and inferior sides of the disc referred to as the cartilaginous endplates (CEP) (Figure 1A). Like most tissues, the precursors to the IVD are highly vascularized during early stages of formation, but most of these blood vessels disappear following early development, leaving the IVD as the largest avascular structure in the body (Humzah and Soames, 1988; Eyre et al., 1991; Christ and Wilting, 1992; Rodrigues-Pinto et al., 2014). As such, the IVD relies on passive diffusion and fluid convection from the vertebral bone through the CEP for nutrient consumption and waste removal (Urban et al., 1977, 1982; Roberts et al., 1989; Urban et al., 2004; Huang et al., 2014). Nutrients take two primary pathways; the blood vessels that surround the outer AF and the embedded blood vessels that terminate at the bony endplate next to the cartilaginous endplates (Holm et al., 1981; Adams and Hutton, 1986; Grunhagen et al., 2006). Passive diffusion is believed to be the leading mode of transport for smaller molecules such as glucose, lactic acid, and oxygen and is influenced by concentration gradients (Rajasekaran et al., 2004; Magnier et al., 2009). Fluid convection transports larger molecules such as growth factors, proteins, enzymes, and hormones and is influenced heavily by hydraulic pressure gradients (Maroudas, 1975; De Geer, 2018).
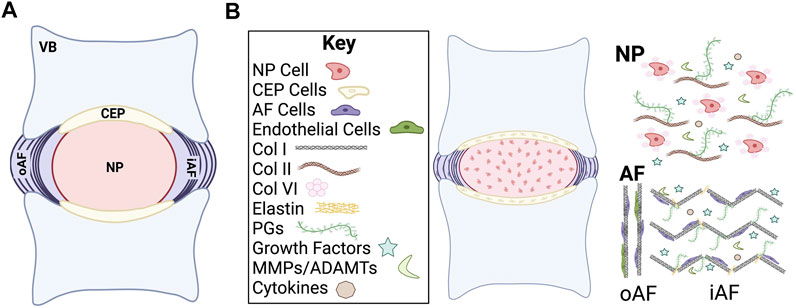
FIGURE 1. Schematic of intervertebral disc (IVD) structure. (A) Midsagittal cut plane of the IVD. (B) Overview of general cellular and chemical composition of the IVD. VB, vertebral body; CEP, cartilage endplate; NP, nucleus pulposus; oAF, outer annulus fibrosus; iAF, inner annulus fibrosus; Col, collagen; PGs, proteoglycans; MMPs, matrix metalloproteases; ADAMTs, a disintegrin and metalloproteinase with thrombospondin motifs.
The IVD is primarily composed of extracellular matrix (ECM) molecules, including multiple types of collagens and proteoglycans (Roughley et al., 2011; Sivakamasundari and Lufkin, 2012; Sivan et al., 2014a) (Figure 1B). The proteoglycans are highly hydrophilic, which allows the IVD to maintain consistent osmotic pressure (Iozzo and Schaefer, 2015; Dou et al., 2021). Collagen type and spatial distribution vary depending on the IVD region but type I and II collagen are the predominant species. Type I collagen and type II collagen are both fibrillar collagens that undergo significant changes in composition and organization as the IVD develops into a load bearing structure, which allow the IVD to withstand internal and external forces that are applied to the spine. The ECM plays a substantial role both in providing physical structural support to the IVD as well as in regulating biological and cellular signals during development.
The main function of the NP, the inner core of the IVD, is to resist compression of the spine and to redistribute the applied load to the spine (Buckwalter, 1995; Lundon and Bolton, 2001). The healthy NP is primarily comprised of highly gelatinous and hydrophilic material that is a combination of negatively charged proteoglycans, collagen, and non-collagenous proteins. Proteoglycans are made up of core proteins that are covalently attached to one or more types of highly anionic glycosaminoglycans (GAGs). The most abundant proteoglycan in the NP is aggrecan, but it should also be noted that decorin, biglycan, fibromodulin, and versican have also been observed (Sztrolovics et al., 1999; Melrose et al., 2001). The NP has a higher concentration of type II collagen as compared to type I collagen. Other key ECM proteins such as elastin, fibronectin, and laminin are also found in abundance in the NP and confer important physical and biological functionality (Yu et al., 2002; Chen et al., 2009).
The main cell types in the NP are categorized as notochordal and chondrocyte-like large vacuolated cells (Roughley, 2004). NP cells have a large diameter (30–40 µm diameter) and exist in the disc as highly organized interconnected cell clusters that allow for cell-cell communication (Pattappa et al., 2012; Hwang et al., 2014). From early development, NP cells have a highly organized cytoskeleton network that is composed of F-actin, microtubules, vimentin, and cytokeratin intermediate proteins (Li et al., 2008). A strong cytoskeleton network plays an integral role in the ability of the NP to withstand the compressive mechanical load and stress. In contrast to developing NP cells, mature NP cells are described as chondrocyte-like, are smaller (∼10 µm diameter), and do not contain intracellular vacuoles (Hunter et al., 2003). In addition to the loss of cellularity in the NP, the water and proteoglycan content also decreases through ageing, leading to a reduction in disc height and nucleus pressurization over time (Bridgen et al., 2013). The transition from notochordal cells to chondrocyte-like cells is currently debated, with some evidence that notochordal cells are completely replaced with chondrocyte-like cells in certain model systems while other studies show retention of a population of notochordal cells past early developmental stages (Minogue et al., 2010; Risbud et al., 2010). Since notochordal cells are highly synthetic, particularly for hydrophilic ECM molecules like aggrecan core protein and GAGs, either complete or gradual loss of this cell population in the IVD likely contributes to increased risk of degeneration over time (Risbud and Shapiro, 2011).
The AF provides support to the gelatinous structure of the NP by encasing the disc in lamellar-structured rings that are mainly composed of organized collagen fibers and some fibroblast-like cells (Sivakamasundari and Lufkin, 2012; Colombier et al., 2014b). These lamellae resist external forces exerted on the IVD components (García-Cosamalón et al., 2010). There are two main regions of the AF, the inner and outer. The inner AF is primarily composed of type II collagen, proteoglycans, and poorly organized ECM. In comparison to the inner AF, the outer AF is highly organized and rich in type I collagen (Colombier et al., 2014a). The cells of the inner AF are morphologically rounder in shape while the cells in the outer AF are more elongated (Williams et al., 2019). This difference in organization is tied to the function since the outer layer provides more resistance to external tension. The cells of the AF are specifically derived from a population of Scleraxis (Scx)+/SRY-related HMG-box (Sox)9+ progenitors that are originally unorganized but eventually align into the lamellar pattern as determined by actin stress fiber networks (Hayes et al., 1999; Sugimoto et al., 2013). This cellular alignment is thought to be critical for the organized formation of the collagen matrix, since the patterning lays the template for the lamellae structure (Torre et al., 2019). AF cells are generally characterized as fibroblast-like elongated cells that are organized in concentric circles around the NP (Peacock, 1951).
Two CEPs are located on the cranial and caudal ends of the IVD. CEPs are comprised of subchondral bone and hyaline cartilage and are the linkage points between the vertebral bone and the AF (Sivakamasundari and Lufkin, 2012). CEPs are rich in type II collagen and proteoglycans with a water content of 50–60%, with chondrocytes making up most of the resident cell population (Roberts et al., 1989). The CEPs allow for the transfer of load that is applied to the IVD and play an important role in the flow of nutrients/waste in and out of the IVD.
Intervertebral Disc Development
During gastrulation, the blastula reorganizes from the single layer of cells into layers of cells that can begin the process of differentiating into germ layers: ectoderm, endoderm, and mesoderm. IVD components arise from the chordamesoderm or paraxial mesoderm. The chordamesoderm gives rise to the notochord and, ultimately, the NP. The paraxial mesoderm gives rise to the sclerotome that forms the vertebrae, CEP, and AF (Colombier et al., 2014b).
Nucleus Pulposus
The nucleus pulposus is composed of cells that arise from the embryonic notochord, a transient midline structure that serves as the primitive axial skeleton in the developing embryo (Figure 2A). Importantly, the notochord is the main signaling center that directs the patterning of the surrounding tissues during early development (Pourquié et al., 1993; Fouquet et al., 1997; Stemple, 2005). Forkhead box A2 (FoxA2), Brachyury (T), and Notochord homolog (Noto) are all proven to be essential for regulating the development of the notochord (Vujovic et al., 2006; Maier et al., 2013). In addition to T-brachyury, expression of Sonic hedgehog (Shh) and hepatocyte nuclear factor (HNF)-3β (also known as FoxA2) is important for successful differentiation and survival of notochordal cells (Risbud and Shapiro, 2011). T-brachyury is mainly restricted to the notochord and tailbud and has been shown to affect the development of the tail and vertebrae (Wilkinson et al., 1990; Pattappa et al., 2012). The transcription factor T-brachyury and adherens junction protein N-cadherin are highly expressed NP cells during early development as compared to mature NP cells or NP cells in a degenerated disc environment (Minogue et al., 2010). FoxA2 primarily affects the development of the node, notochord, and floor plate (Ang and Rossant, 1994). The unique developmental origin of the NP has been confirmed by multiple labs through lineage tracing and mouse model studies as described below (Walmsley, 1953; Hunter et al., 2003; Choi et al., 2008; McCann et al., 2012; Sivakamasundari and Lufkin, 2012; Alkhatib et al., 2018).
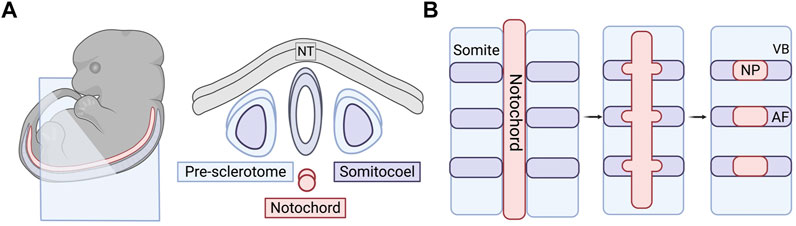
FIGURE 2. Embryonic and early formation of the intervertebral disc (IVD). (A) Embryonic tissues and cell sources that play a role in forming the spinal column. Right panel is a cross-sectional view of the blue cut plane indicated in the embryo in the left panel. (B) Sclerotome and somite condensation in formation of vertebral bodies and the annulus fibrosus. Notochord condenses into distinct bulges along the long axis where the NP will form. The AF tissue surrounds the forming NP over time and the VBs section off in between the individual IVDs. NT, neural tube; IVD, intervertebral disc; VB, vertebral body; AF, annulus fibrosus; NC, notochord; NP, nucleus pulposus.
SHH protein plays a critical role in the patterning of the anterior-posterior axis during embryonic development (Harfe et al., 2004). Using a tamoxifen-inducible Shh-cre mouse model, Choi et al. produced a fate map of SHH-expressing cells in the axial skeleton to track notochordal cells throughout their development (Choi et al., 2008). Through the activation of R26R:eYFP in the notochord, they were able to track the notochord cells via YFP fluorescence and concluded that the majority of the cells become localized to the nucleus pulposi. There are a few notochordal cells that have been found in the spinal column outside of the NP in lineage tracing studies, but it is unclear as to whether this is a result of aberrant localization during development. It has also been conjectured that these minor populations give rise to chordomas later in life. Choi & Harfe expanded on this work by removing the hedgehog signaling at different stages of development (Choi and Harfe, 2011). When signaling was removed from all SHH-expressing cells, the NP was smaller in the mutant mice when compared to the control. The AF also lost the concentric rings, which the authors speculated was a result of the lack of NP development. As the notochord develops, bulges form along the long axis where the IVDs will eventually form the NP (Figure 2B) (Smith et al., 2011; Tomaszewski et al., 2015). In the mutant mice, the notochord was not only thinner, but these bulges never developed. The notochordal cells were instead found all along the length of the entire spinal column rather than localized to disc regions. They also temporally knocked out SHH protein signaling before and after the formation of the notochord sheath. They found that signaling is required before the sheath formation for proper patterning of the spinal column but is not required later in development to maintain structure (Alkhatib et al., 2018).
In a separate study, McCann et al. developed a Noto-cre mouse model to target node and posterior notochord formation during gastrulation (McCann et al., 2012). By examining staining patterns of β-galactosidase and cytokeratin-8 as the embryos developed, they were able to trace the notochordal-derived cells, which independently confirmed that notochordal cells do indeed give rise to the NP. McCann et al. also demonstrated that small chondrocyte-like NP cells were derived from the notochord and were phenotypically distinct when compared to chondrocytes from articular cartilage (Clouet et al., 2009; Minogue et al., 2010). Genes such as Danforth’s short tail (Sd), Shh, smoothened (Smo), Sox5/Sox6, and TSC Complex Subunit 1 (TSC1) were essential for NP development (Lane and Birkenmeier, 1993; Alfred et al., 1997; Choi and Harfe, 2011). In addition to their contribution to the formation of the NP, notochordal cells also aid in the synthesis of ECM during the formation of the spinal column through the stimulation of growth factors (Pattappa et al., 2012).
Annulus Fibrosus
The AF, along with the vertebrae, CEPs, tendons, and ligaments are derived from the sclerotome of the somites (Williams et al., 2019; Bagnat and Gray, 2020). Prior to the specification of the sclerotome, the somites are derived from the paraxial mesoderm through the inhibition of bone morphogenetic protein (BMP) signaling in the lateral plate mesoderm by follistatin, an activin-binding protein, working in tandem with Noggin (Stafford et al., 2014). After the specification and formation of the sclerotome, it undergoes organization and resegmentation where the cells at the caudal boundary of the von Ebner’s fissure, the furrow that divides the rostral and caudal halves of the somite, will develop into the AF or the vertebral body through condensation of the cells (Christ and Wilting, 1992; Christ et al., 2007; Alkhatib et al., 2018) (Figure 2B). The AF will further differentiate into the inner AF, a region that exhibits characteristics of fibrous tissue and cartilage, and outer AF, a primarily fibrous region (Van den Akker et al., 2017).
There are many signaling pathways involved in the determination of the AF. Mesenchyme forkhead 1 (Mfh1) and transforming growth factor (TGF)-β1 are known to play vital roles (Sivakamasundari and Lufkin, 2012). Paired box (Pax) 1 and Pax9 deletions can also affect the sclerotome cell proliferation, leading to a lack of vertebral bodies, proximal portions of the ribs, and IVD (Wallin et al., 1994; Peters et al., 1999; Baffi et al., 2004; Jin, 2011; Mundy et al., 2011). Initial Pax1 and Pax9 expression is seen throughout the AF, but expression eventually becomes enriched in the caudal region later in development (Williams et al., 2019). Additional mouse studies have shown that Indian hedgehog (Ihh), TSC1, and Wnt/βcat are required for AF formation (Maeda et al., 2007; Kondo et al., 2011; Yang et al., 2017; Silva and Holguin, 2020). TGF-β1 plays a pivotal role in the development and growth of the spine, particularly during the differentiation of the sclerotome into cells that eventually form the AF (Gantenbein-Ritter et al., 2011; Stoyanov et al., 2011; Leung et al., 2014). Genes and signaling pathways that play an important role in spinal column patterning and IVD formation are summarized in Table 1.
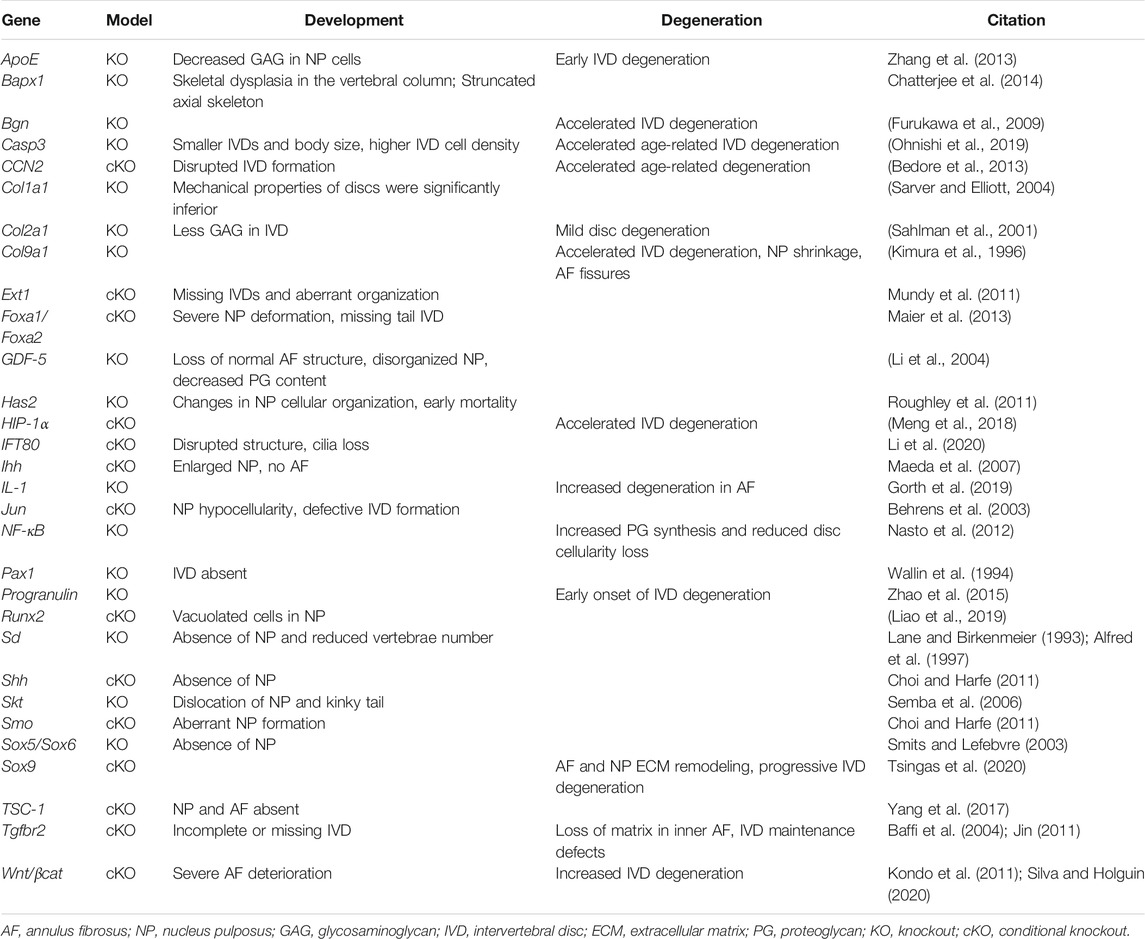
TABLE 1. Summary of genes and signaling pathways that play an important role in intervertebral disc development.
Intervertebral Disc Degeneration
Intervertebral disc degeneration (IDD) is a cell-mediated response to the functional and structural failure of the disc (Adams and Roughley, 2006). Disc degeneration is caused by multiple factors and is associated with ageing, mechanical stress, genetic factors, and nutritional disorders. Mechanical stress can arise from hypermobility and overloading of the disc in the early stages of IDD, which is then generally followed by limited motion and hypomobility in the later stages (Stokes and Iatridis, 2004). Other physical factors that contribute to IDD include obesity, high BMI, bad posture, and smoking (Fogelholm and Alho, 2001; Hangai et al., 2008; Elmasry et al., 2015; Sheng et al., 2017). IDD can lead to many related and often progressive medical conditions as a result of spinal instability, disc herniation, and nerve impingement such as spondylosis, which refers to degenerative and osteoarthritic changes to the discs and vertebral bones, and sciatica, where pressure put on the sciatic nerve root by a herniated disc or bone spur can cause discomfort, pain, and limb weakness (Mulleman et al., 2006; Ferrara, 2012).
Although IDD is a multifactorial disease, recent studies have shown that genetic factors play a critical role in the onset and progression of IDD (Feng et al., 2016). Population studies have indicated polymorphisms in genes such as COL1A1, COL9A2, COL11A1, Aggrecan, and IL-1 may play a role in disc degeneration (Annunen et al., 1999; Kawaguchi et al., 1999; Solovieva et al., 2004; Tilkeridis et al., 2005; Mio et al., 2007; Janeczko et al., 2014; Yang et al., 2019). Due to limitations in collecting human patient samples, very few genome-wide association studies (GWAS) in humans have been completed (Urban and Fairbank, 2020). Human GWAS meta-analyses have found novel candidate genes that, when mutated, may be associated with disc degeneration, including PARK2 (Williams et al., 2013) and CHST3 (Song et al., 2013). The GWAS analysis that identified PARK2 involved 4,600 northern European individuals with IDD and suggested that methylation of the PARK2 promoter may induce IDD. The analysis that identified CHST3 as a candidate gene in disc degeneration involved a total of 32,642 human subjects of Southern Chinese descent, with 4,083 individuals with lumbar disc degeneration and 28,599 controls. This study found that mutations in CHST3 altered synthesis of ACAN, one of the crucial proteoglycans responsible for disc hydration. Meta-GWAS analysis of 158,000 individuals of European descent identified 3 novel loci; rs12310519 in SOX5, rs4384683 in CCDC26/GSDMC, and rs4384683 in DCC, that may be associated with chronic back pain (Suri et al., 2018). In addition to the three above mentioned loci, a follow up study by Freidin et al. also found that mutations in SPOCK2 and CHST3 potentially contribute to disc generation and general back pain in a study of 509,000 European individuals (Freidin et al., 2019). However, there is debate as to whether the SOX5, CCDC26/GSDMC, and DCC loci are associated with back pain based on another study of Caucasian individuals in Norway (Lie et al., 2022).
Reduction and long-term loss of nutrition also appear to be key factors responsible for disc cell changes (Buckwalter, 1995; Urban et al., 2004). Blood vessels in the disc margins supply the disc with essential nutrients and substrates (Eyre et al., 1991). Ageing and prolonged mechanical load can damage the CEPs, impairing nutrient delivery and waste removal from the IVD, resulting in disc tissue breakdown (Boos et al., 2002). Increasing age and degeneration also result in disorganization of the disc morphology. This includes disorganization of the annular lamellae with decreased proteoglycan content, bifurcation, and interdigitation (Lai et al., 2015). While increased cell proliferation as a response to damage and stress has been observed (Johnson et al., 2001), there seems to be a concomitant increased senescence and apoptosis (Gruber and Hanley, 1998; Roberts et al., 2006).
ECM homeostasis is maintained through catabolic and anabolic regulation and is essential for the structural integrity of the IVD (Moon et al., 2014). In IDD, there is increased expression of matrix-degrading proteins such as matrix metalloproteinases (MMPs) and a disintegrin and metalloproteinase with thrombospondin motifs (ADAMTs) resulting in deregulation of the normal homeostatic mechanism (Le Maitre et al., 2007). The loss of proteoglycans is a major biochemical change observed in IDD. As aggrecan becomes degraded, associated GAGs are lost, which leads to loss of hydration in the NP (Sivan et al., 2014b; Wei et al., 2019). This reduction of NP hydration results in loss of disc height and disc bulging (Kumaresan et al., 2003). Degenerating discs also show loss of collagen fibers and increase in fibronectin (Hollander et al., 1996; Oegema et al., 2000).
Oxidative inflammation is also thought to be a major cause of molecular damage through various stressors (McGarry et al., 2018). While IVDs reside in a low oxygen tension environment, reactive oxygen species (ROS) are generated through oxidative phosphorylation (Ali et al., 2008). ROSs damage lipids, DNA, and proteins, and affect the structural and functional homeostasis of the disc (Feng C. et al., 2017).
Several animal models, such as sand rats and Chinese hamsters exhibit naturally occurring IDD (Silberberg and Gerritsen, 1976; Adler et al., 1983; Moskowitz et al., 1990), but in consideration of consistency and time-constraints in IVD research, multiple disc degeneration models have been generated using physical, chemical, or genetic modification (Singh et al., 2005). Specifically, SM/J mice, known for their poor regenerative capacities, displayed early spontaneous onset IDD that was marked by early onset cellular and matrix changes that were consistent with human IDD, such as loss of NP/AF boundary, formation of small clusters in the NP, and chondrocyte-like cells surrounded by proteoglycan-rich matrix (Choi et al., 2018). Furthermore, in a comparative study of SM/J mice and LG/J mice, a strain known for its super tissue healing capacity, Zhang et al. reported that changes in the NP affected ion regulation within this disc region, which led to dysregulated cellular homeostasis and fibrotic changes to the tissue (Zhang et al., 2018).
Physical methods to induce degeneration have included protocols such as forelimb amputations to force bipedalism, annulotomies, and endplate injury (Yamada, 1962; Stern and Coulson, 1976; Lipson and Muir, 1981; Higuchi et al., 1983; Holm et al., 2004). Chemical methods include chemonucleolysis, fibronectin fragment injections, and smoking (Bradford et al., 1983; Simmons et al., 1984; Holm and Nachemson, 1988; Iwahashi et al., 2002; Greg Anderson et al., 2003). One of the more commonly used methods to induce disc degeneration in recent studies has been the needle puncture injury method in both in vivo and ex vivo small and large animal models, where the IVD is punctured using needles of various gauges (Korecki et al., 2008; Michalek et al., 2010; Martin et al., 2013; Piazza et al., 2018). The severity of degeneration can be varied based on the location and depth of the puncture and size of the needle used (Moriguchi et al., 2018; Yang et al., 2018; Qian et al., 2019). The caudal IVD is frequently targeted for this method due to the ease of access as the caudal spine lacks transverse and posterior processes and does not encase the spinal cord. While the needle puncture injury method provides reliable and consistent experimental disc degeneration models, findings from these studies need to be carefully interpreted and considered in a context dependent manner as the animal caudal spine differs in anatomy, mechanical load, and degrees of motion as compared to the human spine.
Non-rodent animal models for IVD include rabbits, which display a similar disc morphology to humans and have been used to study degeneration as well as potential therapeutics (Kroeber et al., 2002; Liu et al., 2011; Calvo-Echenique et al., 2018). Multiple larger animal models have also been established including canine, ovine, caprine, camelid, and porcine models (Reid et al., 2002; Yoon et al., 2008; Bergknut et al., 2012; Stolworthy et al., 2015; Gullbrand et al., 2017a). Due to their similarity to humans, non-human primates have been used as models for IVD research and therapeutics. Although they are usually quadrupedal, primates such as baboons and macaques spend significant time in erect positions and display spontaneous disc degeneration (Lauerman et al., 1992; Platenberg et al., 2001; Gal, 2002; Nuckley et al., 2008). Rhesus monkeys have been utilized as they display age-related IDD with comparable histologic pathology to human disc degeneration (Bailey et al., 2014). Baboons and rhesus monkeys have also been used to analyze the efficacy of IVD prostheses and autograft surgeries (Luk et al., 1997; Cunningham et al., 2002; Luk et al., 2003; McAfee et al., 2003). Non-human primates and large animal models are particularly useful models as their discs are similar in size and morphology to humans. Primates also face biomechanical stress from an upright position that cannot be replicated in quadrupedal animal models. However, their usage involves extensive ethical considerations, and the high costs and practical requirements can restrict their use in many studies.
Pain Management in Current IDD Therapies
Although there are currently no cures for IDD and back pain, there are multiple chemical treatments that are used to manage IDD and discogenic pain. The initial standard approach to treating lower back pain is a 4–6-weeks conservative therapy course involving analgesics, NSAIDs (Non-steroidal anti-inflammatory drugs), and physical therapy (Masala et al., 2019). If there is no improvement or relief from this treatment, percutaneous procedures are utilized. Percutaneous procedures serve as an intermediate step between conservative therapy and decompression and surgical options (Masala et al., 2019).
There are a variety of percutaneous procedures that can be classified into three categories: annuloplasty, percutaneous disc decompression, and endoscopic percutaneous discectomy. Annuloplasties are procedures aimed at repairing the disc annulus (Kapural and Houra, 2012). Intradiscal electrothermal therapy (IDET), a form of annuloplasty, is a minimally invasive surgical procedure where heat is applied to the AF through a catheter, preventing future ruptures through the change in collagen structure and burning of nociceptors to decrease the level of pain (Saal and Saal, 2000a; Saal and Saal, 2002b). Radiofrequency is also frequently utilized to treat discogenic pain with methods such as radiofrequency annuloplasty (RFA), which is a minimally invasive method that delivers radiofrequency thermal energy to the disc to denervate the AF and provide pain relief (Gelalis et al., 2019). Intradiscal radiofrequency thermocoagulation (IRFT) involves the direct application of radiofrequency energy to the center of the disc (Troussier et al., 1995). Another annuloplasty method is the intradiscal biacuplasty, which involves placing two radiofrequency electrodes on the posterolateral sides of the annulus fibrosus (Kapural and Mekhail, 2007).
Despite the theoretical benefits of these treatments and their extensive use, it appears that they have little clinical advantage over sham procedures (Kepler et al., 2011). One clinical study involving 57 patients suffering from chronic discogenic lower back pain (CDLBP) observed the effects of IDET (38 patients) or a sham procedure (19 patients) and reported no significant improvement in either group (Freeman et al., 2005). Another study involving 28 CDLBP patients compared the clinical outcomes of IRFT (13 patients) to a placebo treatment (15 patients) and found that IRFT was not effective in reducing CDLBP (Barendse et al., 2001). However, it should be noted that both of these trials were conducted on a small sample size and further large-scale clinical trials are needed to provide more information on the clinical relevance of these treatments.
Other percutaneous procedures targeting herniation and discogenic pain include endoscopic discectomy and disc decompression that includes laser discectomy, plasma discectomy, and mechanical disc decompression (Raj, 2008). Endoscopic discectomy, which endoscopically removes a portion of the NP, is a good therapeutic treatment for discogenic pain and disc protrusion (Xu G. et al., 2020). Percutaneous lumbar laser discectomy involves using laser energy to vaporize a small volume of the NP resulting in reduced pressure; however, there is limited evidence of the clinical benefits (Singh et al., 2013). Currently there are commercial decompression systems such as the Dekompressor or high RPM devices available for percutaneous discectomies. The device is a high rotation device that extracts nuclear material through a cannula. A review found limited evidence for the use of the Dekompressor; however, due to the small number of both randomized or observational trials, further research has to be conducted on the method (Manchikanti et al., 2013). Overall, despite their widespread use over the years, it appears that multiple percutaneous disc procedures do not have significant clinical relevance in the treatment of IDD and discogenic pain.
Chemonucleolysis is a form of non-surgical treatment for discogenic pain and herniated discs (Alexander, 1986), which involves the administration of a proteolytic enzyme like chymopapain into the IVD to alleviate fibrotic tissue damage and reduce disc bulging (Thomas, 1956). Unfortunately, the long-term effects of chemonucleolysis includes lower back pain partially due to depolymerization of the overall NP structure and loss of water content. Due to these adverse effects, the technique is no longer used in the United States and Europe. A newer version of this treatment uses a more specific GAG-degrading enzyme condoliase (Chiba et al., 2018; Matsuyama and Chiba, 2019; Muramatsu et al., 2020).
As conservative therapy and percutaneous procedures are quite palliative in nature and may restrict functionality, surgery is utilized to help alleviate discogenic pain and with the hope of providing better mobility and function. Surgical treatments may include IVD excision, spinal fusion, or artificial disc replacement (Bowles and Setton, 2017). Spinal fusion is a commonly used surgical procedure to treat IDD that involves joining two or more vertebrae using bone grafting (Tehranzadeh et al., 2005). Spinal fusion has many advantages including elimination of motion at the degenerated disc, allowing for better decompression and elimination of instability. Currently, bone grafts are obtained from the patient themselves (autograft), a consenting donor (allograft), or using artificial bone substitutes. To eliminate the morbidity relating to autografts and allografts, there has been extensive development of artificial substitutes (Gupta et al., 2015). Artificial bone substitutes have been created from natural materials such as demineralized bone matrix substitutes and ceramic substitutes, originally obtained from corals (Girardi and Cammisa, 2003; Chen et al., 2005). Due to the recent advances in tissue engineering, there is also promise of using biomaterials such as hydrogels and nanofiber scaffolds as synthetic bone substitutes. The possibility of being able to release controlled growth factors from the biomaterial during spinal fusion also makes biomaterials an attractive solution (Gupta et al., 2015).
Despite hundreds of thousands of individuals receiving spinal fusion surgery annually, there are various factors that bring into question the clinical significance and efficacy of the procedure. A study observed that for nonradicular back pain, fusion surgery was no more effective than intensive rehabilitation (Chou et al., 2009). An analysis of patient satisfaction indicated that on average 68% of patients were satisfied after spinal fusion surgery with frequently reported complications including pseudoarthrosis (14%) and chronic pain at the graft site (9%) (Turner et al., 1992). A large clinical study in South Korea of 629 patients who underwent spinal fusion also found that post-operative patient satisfaction was around 71%, with unsatisfied patients reporting the persistence of chronic pain after the surgery (Kong et al., 2010). Analysis of a cohort of 874 patients who underwent lumbar spinal fusion found that at 24 months post-surgery, an alarming 44.4% of patients had been prescribed opioids for continued pain management and 37.8% of patients were receiving mental health treatment for stresses related surgery and back pain (McMillan et al., 2021). Additional studies have shown that spinal fusion may result in degeneration of adjacent discs and lead to further discogenic pain, herniation, stenosis or spondylosis, suggesting that improvements in surgical interventions for back pain are necessary (Ghiselli et al., 2004).
As spinal fusion surgery may limit mobility, artificial discs or cervical disc replacement (CDR) devices have emerged as an alternative as they preserve range of motion (Greg Anderson et al., 2003). As of 2020, there are 15 artificial discs actively used in global markets with 7 of them approved for use in the United States (Turel et al., 2017; Nunley et al., 2018). Clinical studies have demonstrated that artificial disc replacements are a viable alternative to spinal fusion or percutaneous procedures and are superior in terms of neurological success and range of motion (Heller et al., 2009; Gao et al., 2015). Studies have also shown that the clinical outcomes may differ among various CDR devices, requiring surgeons to tailor the device choice to specific patient profiles (Wahood et al., 2020). Complications from disc replacement can include incomplete decompression, malposition, infection, and vertebral body fracture (Steinberger and Qureshi, 2020). Despite their benefits, due to the technical challenges of the surgical procedure, the high cost, and outcomes similar to spinal fusion, total IVD disc replacements are not yet widely used (Phillips et al., 2015).
Development of Regenerative Strategies and Therapeutics
Due to limitations of current treatments, there has been emerging interest in the treatment of IDD using cell-based and biomaterial engineering therapies as summarized below. Preclinical and clinical studies demonstrate potential for the treatment of disc degeneration using cell-based therapies (Sakai and Schol, 2017; Urits et al., 2019). Currently ongoing and recently completed clinical trials are summarized in Table 2. Many clinical trials have focused on injection of a combination of growth factors, biomimetic materials, and cultured cells with varied success. However, due to small sample sizes and lack of comparative studies, extensive research needs to be conducted before the widespread introduction of these treatments (Meisel et al., 2019). Furthermore, as cell-based therapies primarily involve implantation of stem cells, the source of these cells can lead to ethical issues and treatment scrutiny. Currently, most clinical trials utilize autologous stem cells that are expanded in an in vitro tissue culture setting prior to use for treatment. However, as autologous stem cells are not always available for harvesting dependent on disease severity and presence of comorbidities in patients, some trials are exploring the use of allogeneic cells. This requires additional testing for immunologic and inflammatory responses, potential transmittable diseases, as well as additional consenting steps from the donors prior to implantation. The ethical and legal considerations for stem cell treatments and clinical trials are also dependent on each individual country’s healthcare and biomedical legislations, further complicating widespread implementation of cell-based therapeutics (Dhar and Hsi-En Ho, 2009). Further complicating the strategy of directing cellular differentiation into healthy IVD tissue is the unique developmental origin of the NP. As discussed previously, unlike most of the surrounding tissue that is of mesenchymal origin, the NP arises from the notochord, which is a transient structure. In some animals, especially rodents, there is evidence of molecularly distinct notochordal cells that persist in the NP well past the early developmental stages. However, this cell population disappears at early neonatal stages in humans, and thus, there is no good natural cellular depot from which these cells can be harvested and cultured in human patients. Therefore, a significant challenge in cell-based NP regeneration therapies is defining the unique cellular genotype and phenotype of the progenitor notochordal cells and recapitulating this distinct cellular profile in cells of non-notochordal origin.
Healthy NP tissue generally contains two cell types, depending on age and species: notochordal cells that play an important role in IVD development, growth, and homeostasis, and the nucleopulpocytes that maintain the IVD post development (Clouet et al., 2019). Studies have shown that mouse induced pluripotent stem cells (iPSCs) have the potential to differentiate into NP-like cells (Chen et al., 2013). Based on developmental studies, notochordal cells could be a tool for regenerative medicine. Given their early embryonic origin and limited numbers, they will likely need to be generated from iPSCs or ESCs. One study demonstrated that human iPSCs could be differentiated into notochordal cell-like cells when cultured in the presence of natural NP tissue matrix (Liu et al., 2014; Liu et al., 2015). A separate study focused on the NOTO and NOTO-related genes’ roles in the commitment of mesendoderm progenitor cells towards a notochordal fate by maintaining an expression of the regulatory factors FoxA2 and T-brachyury (Colombier et al., 2020). Notably, Zhang et al. established a NOTO-eGFP reporter in a human pluripotent stem cell (hPSC) line using CRISPR/Cas9-mediated genome editing that was verified by comparing them to two human NP cell transcriptome datasets (Zhang et al., 2020). Currently, human PSCs have limited clinical utilization as rapid growth and safety are of major concern (Hynes et al., 2014; Clouet et al., 2019). However, a new method has been devised to efficiently differentiate human PSCs toward NP-like cells, which may be able to provide a cell source for future regenerative therapies (Tang et al., 2018). Tang et al. developed various chemically defined mediums and growth factor supplementation to direct undifferentiated human PSCs to an NP-genic phenotype through a stepwise differentiation. Early exposure to Activin A and Wnt-3a, followed by varying levels of exposure to BMP4 and FGF2 induced cell differentiation through a mesodermal and notochordal lineage to NP-like cells.
Another potential cell source for cell-based therapy to treat IDD is mesenchymal stem cells, also known as mesenchymal stromal cells (MSCs). MSCs can be derived from robust sources like the bone marrow and adipose tissue, even from adult donors, and escapes much of the ethical and safety scrutiny that ESCs undergo in cell-based therapeutics development (Pittenger et al., 1999; Zuk et al., 2001; Blanco et al., 2010). Animal model studies demonstrated that MSCs injected into the NP can survive for months and proliferate under specific conditions (Hiyama et al., 2008; Henriksson et al., 2009; Shen et al., 2015). Further research has indicated that certain growth factors such as growth differentiation factor (GDF) 5 and GDF6 could induce adipose-derived MSCs (ASCs) to differentiate into NP-like cells (Stoyanov et al., 2011; Clarke et al., 2014; Colombier et al., 2016). Transplantation of MSCs using a hydrogel or colloidal gel carrier also increased the regenerative capabilities (Pereira et al., 2014; Chen et al., 2019; Choi et al., 2020; Wang Y. et al., 2021). In a human trial, injections of autologous expanded bone marrow MSCs into the NP resulted in elevation of water content and rapid improvement of pain (Orozco et al., 2011). While MSCs may be an attractive alternative source to ESCs in cell-based therapies, difficulty in continued proliferation in vitro, off-target differentiation into other cell types, fibrotic changes, and cellular senescence are issues that have yet to be fully addressed.
Recent advances in polymer and chemical engineering have led to the rapid development of synthetic and 3D printed bioimplants and hydrogels (Bowles and Setton, 2017; Shi et al., 2020; Li et al., 2021; Yan et al., 2021). Recently developed hydrogels and biomaterials have been summarized in Supplementary Table S1. In regenerative therapeutics, hydrogels and biomaterials are primarily used as scaffolds to support the growth and cell viability of cell-based treatments such as MSC injections. Biomaterial scaffolds can be used in combination with cell-based therapies to support NP regeneration and serve as carriers for cell and vector delivery (Li et al., 2016; Priyadarshani et al., 2016). Nanofiber material such as sponge microspheres (NF-SMS) mimic the fibrous ECM environment whereas materials such as polycaprolactone (PCL) and poly(ether carbonate urethane)urea (PECUU) are used for scaffolds that mimic the biomechanical properties of natural tissue (Zhang et al., 2015; Zhu et al., 2016; Christiani et al., 2019). Synthetic hydrogels, which are 3D network microstructures, show great potential with hydrogels like polyvinyl alcohol (PVA) and polyvinylpyrrolidone (PVP) having similar biomechanical properties to human discs (Katta et al., 2007). Natural materials such as chitin, gelatin, and alginate have also been used to produce hydrogels like chitosan and gelatin methacryloyl (GelMA) that act as scaffolds and promote ECM production (Guo et al., 2014; Li et al., 2017; Yang et al., 2020). Hydrogels and 3D printed implants to replace the damaged NP have shown potential to restore disc height and reduce pain (Schmocker et al., 2016; Perera et al., 2021).
Omics in IVD Development, Homeostasis, Degeneration, and Therapeutics
The advent of many omics techniques such as whole-transcriptomic sequencing (RNA-Seq), proteomics, and metabolomics have revolutionized our understanding of IVD development by allowing researchers to generate global profiles of gene expression and molecular level changes that are occurring at different time points during IVD formation. Omics studies have added much depth to our knowledge of processes involved in IVD development and helped to identify disc specific makers, as well as led to a better understanding of pathways involved in the maintenance and homeostasis of healthy tissue and degenerative changes that occur in IVD pathology. Some of the major omics investigations are discussed in this section and summarized in the omics tables (Figure 3 and Table 3).
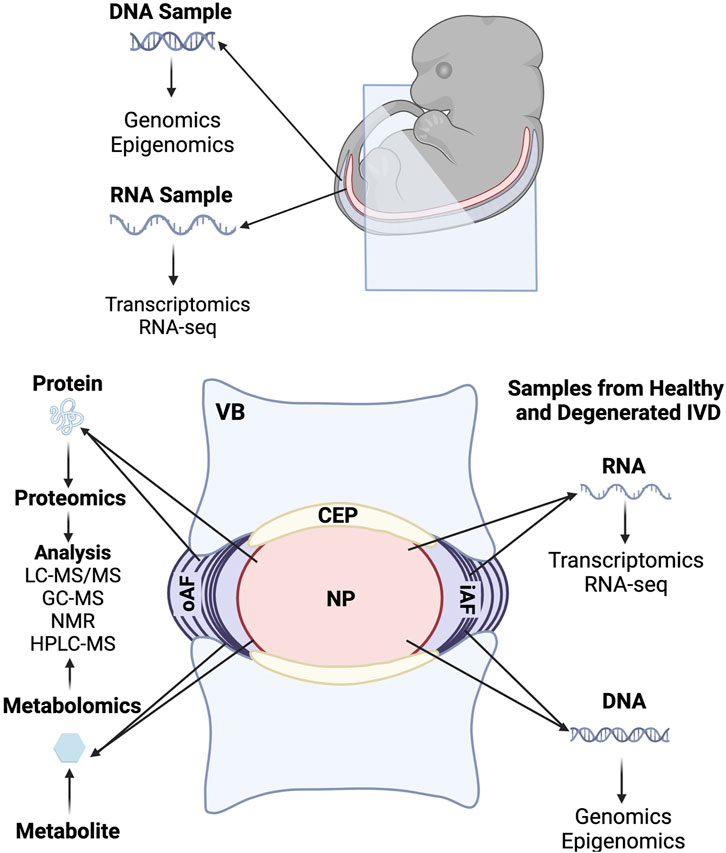
FIGURE 3. Summary of omics investigations carried out during embryonic development and in the fully formed intervertebral disc.
Expression of PAX1 and PAX9 protein is necessary for spinal column patterning and IVD differentiation (Neubüser et al., 1995). Transcriptomics analysis of transgenic embryonic mouse cells showed that Pax1 and Pax9 positively regulate many genes associated with cartilage formation and activate expression of chondrogenic genes during early IVD differentiation (Sivakamasundari et al., 2017). The transcription factors, Sox5, Sox6, and Sox9, known as the Sox trio, all contribute to embryonic skeletogenesis and control chondrocyte differentiation and maturation (Lefebvre, 2019). In particular, Sox9 has been shown to play a major role in the commitment of progenitor cells to the chondrogenic fate. Heterozygous mutations in and around Sox9 can lead to campomelic dysplasia, a severe form of human chondrodysplasia that affects development of the skeleton, reproductive system, and face (Foster et al., 1994; Wagner et al., 1994). Sox9 has also been shown to play a specific role in inner AF differentiation during disc development (Bi et al., 1999). Transcriptomics analysis of Sox9 mutant mice showed that deletion of Sox9 resulted in progressive disc degeneration and alterations in ECM organization indicating that Sox9 expression can also directly impact disc cell survival and ECM maintenance (Tsingas et al., 2020). Mammalian homeobox gene Bapx1 (Nkx3.2) was shown to suppress hypertrophic differentiation of chondrocytes in conjunction with Sox9 since they regulate similar pathways in the same tissues (Chatterjee et al., 2014). Ultimately, Chatterjee et al. found that Bapx1 and Sox9 co-regulate 137 genes that are involved in chondrocyte differentiation.
Shh expression, previously identified to be critically important for embryonic notochordal cell differentiation, was found to be significantly downregulated in early postnatal NP cells (Peck et al., 2017). Using RNA-Seq with the Shh-Cre mouse model, Peck et al. found patterning signals like Shh expression were downregulated over time while expression of synthetic genes like TGF-β and insulin growth factor (IGF) were upregulated in the same developmental window, providing insights into gene families required for patterning, ECM synthesis, and maintenance of the NP.
In addition to extensive transcriptomics studies in mouse models, RNA-Seq studies of isolated human embryonic and fetal notochordal cells have identified temporally specific notochord markers such as CD24, stathmin-2 (STMN2), and reticulon 1 (RTN1), and implicated genes involved in inhibition of vascularization and inflammation as important master regulators of notochordal cell gene expression (Rodrigues-Pinto et al., 2018). Signaling network analysis on healthy human IVD cells also found that platelet-derived growth factor (PDGF) and TGF-β cascades are necessary cues in the formation and maintenance of the NP microenvironment (Gan et al., 2021). A single-cell RNA-Seq (scRNA-Seq) study analyzed primary NP and AF cells isolated from healthy adult discs and observed that receptor signaling pathway genes were highly expressed in the AF, whereas protein synthesis and ECM genes were highly expressed in the NP (Fernandes et al., 2020). In this study, Forkhead box M1 (FOXM1) and lysine demethylase 4E expression (KDM4E) were identified as signature gene markers for the AF and NP respectively. Furthermore, a membranome-based approach on immortalized NP and AF cell lines established from adolescent healthy disc tissue yielded various membrane-associated markers in the AF and NP (van den Akker et al., 2020).
While bulk RNA-Seq has provided key insights into global gene expression profile changes that occur during the development of the IVD, it is important to account for the significant cellular heterogeneity that is present in fully formed IVD. Thus, single cell analyses such as single cell RNA-Seq (scRNA-Seq) can deconvolute some of these differences that may be lost when averaging the entire cellular population, especially at later time points (Kraus et al., 2019). Kraus et al. highlighted the importance of single-cell sequencing through the plate in situ hybridization (PISH) assay, where the cells were first quantified using a population average then assessed using single-cell analysis. They were able to detect differences between the NP, AF, and transition zone in between the NP and AF. Furthermore, the center region of the NP exhibits additional heterogeneity as indicated by the presence of a distinctive set of transcripts that are involved in cell pluripotency (Sox2 and Oct4) and the development of the notochord (Noto and T) and axial skeleton (Shh and Gli3).
Transcriptomics has also been used to identify biomarkers and potential therapeutic targets for IDD. One study analyzed the rat IVD transcriptome using scRNA-Seq and established a comprehensive single-cell gene expression map as well as potential new genetic markers for IVD and multi-functional stem cells (Wang J. et al., 2021). Due to their similarity in size and morphology to human IVD, bovine discs have been popular experimental models in IVD research. RNA in situ hybridization of adult bovine coccygeal IVDs identified 10 novel biomarkers, and further scRNA analysis of bovine calf coccygeal IVDs revealed 27 NP specific genes, 24 AF specific genes, and various cell population clusters (Li et al., 2019; Calió et al., 2021) (biomarkers and genes listed in Table 3). scRNA analysis of bovine calf caudal IVDs identified multiple biomarkers and 15 unique cell clusters of potential IVD progenitor cells (Panebianco et al., 2021). Analysis of notochordal cells derived from human embryonic stem cells identified a regulatory network for notochordal differentiation (Diaz-Hernandez et al., 2020). RNA-Seq of NP samples from human adult patients with IDD was used for genome-wide identification of long noncoding RNAs (lncRNAs) and competing endogenous RNAs (ceRNA) that may serve as diagnostic and therapeutic biomarkers (Zhao et al., 2016). Degenerative disc sequencing on adult human tissue also identified ECM regulatory networks and observed that ECM related genes were associated with multiple growth factors (Riester et al., 2018).
Proteomics is used to identify and quantify proteins in a cell, tissue, or organism and to understand the structure and functions of these proteins (Aslam et al., 2017). Using proteomics technologies, researchers have made great inroads into understanding and optimizing animal models of IVD development, maintenance, and degeneration. Comprehensive proteomic profiles of the healthy mouse IVD have been established using mass spectrometry methods to identify differences in protein expression between the NP and the AF (McCann et al., 2015; Kudelko et al., 2021). Difference gel electrophoresis (DIGE) analysis of the NP from young bovine caudal discs also suggested that subpopulations of notochordal-like cells remain in the mature bovine discs and that these cells may play a role in disc repair and survival (Gilson et al., 2010). These datasets have also been compared to protein expression profiles from human disc samples to assess the relevance of mouse and bovine models for IVD research. Liquid chromatography tandem mass spectrometry (LC-MS/MS) conducted on human fetal IVD samples also identified 10 protein clusters and proteome networks involved in IVD growth and development (Rajasekaran et al., 2020). Proteomics analyses have also established differential expression of proteins between normal and degenerative discs to identify potential therapeutic targets. One study utilized electrophoresis, mass spectrometric analysis, and database research to compare the protein expression profiles of normal and degenerated human AF and found 10 proteins that had highly altered expression between the two groups (Ye et al., 2015). A later study using isobaric tag for relative and absolute quantitation (iTRAQ) and one-dimensional gel electrophoresis-Fourier transform mass spectrometry/ion trap tandem mass spectrometry (FTMS/ITMSMS) that compared normal and degenerative discs showed that in patient samples, 73 proteins in the AF and 54 proteins in the NP exhibited differential expression levels between the two groups (Sarath Babu et al., 2016).
Notably, Tam et al. were able to establish a spatiotemporal proteomics atlas of human intervertebral discs, DIPPER, through various measurement types including label-free proteomics, transcriptomics, and SILAC to degradome (Tam et al., 2020). Two cadaver lumbar samples from a young and an aged cadaver were used to establish a high-resolution point-reference map of static spatial proteomes along the lateral and anteroposterior directions of IVDs at three lumbar levels. They also determined that the point-reference genome-wide profile has clinical relevance since it integrates with the dynamic proteome and transcriptome of clinical samples. They showed that the IVD is comprised of 3,100 proteins, encompassing roughly 400 matrisome proteins and 2,700 non-matrisome proteins, with 1,769 proteins detected in three or more profiles. When this dataset was analyzed, they were able to conclude that the different regions of the IVD (NP, NP/inner AF transition zone, inner AF, AF, and outer AF) had unique protein profiles in healthy IVDs. These profiles will change as the IVD ages, most notably the inner AF becoming gradually similar to the outer AF. Kudelko et al. also performed extensive and comprehensive proteomic profiling in murine models that mimicked a healthy young adult IVD to generate PRIMUS (Proteomic Resource for Intervertebral disc of MUS musculus) (Kudelko et al., 2021). Their findings were consistent to that of the DIPPER data set, where they noted that both the AF and NP have unique profiles. Structurally, the AF in mice and humans are similar since the organized lamellar structure is preserved. In contrast, the NP in mice have a highly cellularity, while the human NP have a greater proportion of ECM.
Both transcriptomic and proteomic profiles of the developing disc have established the unique origin of the NP in mouse and human samples. However, the substantial structural changes that occur in the development of the IVD and the inherent heterogeneity of the fully formed NP, including the differences in cell population between mouse and human samples have made distinctly defining the ultimate mature NP cell profile a difficult task. In a recent study in adult human NP cells, Richardson et al. focused specifically on measuring putative notochordal and NP cell markers and found expression levels of previously identified novel NP and notochordal markers such as KRT8, KRT18, KRT19, CA12, FOXA2, and NOG stayed consistent regardless of donor age or degeneration (Richardson et al., 2017). Additional investigations verifying the persistence of expression of previously identified markers of NP cells throughout development and ageing and large-scale comparative studies between animal and human notochordal/NP cell omics datasets will lead to the establishment of a consensus list of mature NP cell markers in the future.
In addition to well-established transcriptomic and proteomic studies, other types of large-scale omics investigations are gaining momentum in IVD research as technologies become further refined, particularly in important mouse model studies (Veras et al., 2020). Matrisome and metabolome-centered inquiries are examples of such analyses. The matrisome is a collection of genes encoding for the ECM and ECM-associated proteins and allows for better understanding of both development and degeneration (Hynes and Naba, 2012; Naba et al., 2016). One study utilized iTRAQ LC-MS/MS analysis on fetal, young, and old bovine discs to define the NP matrisome (Caldeira et al., 2017). The study found an enrichment of collagen types XII and XIV in fetal discs and fibronectin and prolargin in aged discs and provided a useful database for reference in understanding development and identifying therapeutic targets in the context of the IVD ECM. Matrisome analysis on degenerated adult human samples showed fibrosis-like changes in the ECM due to ageing and degeneration as well as decreased solubility and changes in fibril diameter (Yee et al., 2016). Degeneration also resulted in the upregulation of collagen biosynthesis pathways and genes associated with tissue development and cell death regulation (Rajasekaran et al., 2021).
Metabolomics is the utilization of advanced analysis techniques to identify and characterize metabolites from cells and tissues (Wishart, 2019). Metabolomics have been conducted on human degenerative tissues to identify the changes that occur in pathologic conditions to biological metabolic mechanisms (Swank et al., 2020). One study conducted high-resolution magic angle spinning nuclear magnetic resonance (HR-MAS NMR) spectroscopy on degenerated adult human lumbar discs and demonstrated a correlation between changing metabolite concentrations and degrees of degeneration (Radek et al., 2016). Identifying pathologic alterations to metabolites will be applicable to the development of novel diagnostics and therapeutics. As a corollary to omics studies, large clinical data analyses and artificial intelligence (AI) networks for analyzing large scale, global patient population data on low back pain are important disciplines that have garnered much interest in recent years and continue to advance with the advent of more sophisticated analysis methods. Studies have shown that lower back pain is highly prevalent among the elderly population and causes more global disability than any other condition (Hoy et al., 2014; de Souza et al., 2019). Population studies have also been used to develop prediction models and risk scores for lower back pain (Mukasa and Sung, 2020).
Discussion
The IVD is an indispensable structure in the spine. The three substructures within the IVD work in concert to provide both mechanical support and biological function in the spinal column. The aggregated efforts of many labs over several years have established a solid framework for our understanding of the IVD. As a field, we have made great strides into elucidating many mechanisms that drive IVD development as well as mechanical and biological stresses that lead to IDD and related pathogenesis. While many early genetic and lineage tracing investigations laid the critical foundation for this field, advancements in omics techniques in more recent years have provided global overviews into early embryonic development, the genetic and molecular profile of a healthy IVD vs. a degenerated IVD, and importantly, new strategies in diagnostics and potential disc regeneration therapies.
The complexity of the IVD, in development, composition, and function, makes this unique structure a particularly difficult one to fully understand. As the majority of the human population globally will be afflicted by disc degeneration and associated pain and other complications in their lifetimes, it is critically important to establish thorough and comprehensive molecular profiles of the IVD at various stages of development and disease. With the application of advancing omics technologies to both animal models and human samples, we already have an unprecedented and detailed view of many aspects of the IVD. While animal models are by no means a perfect replication of the human disc due to differences in IVD size, cell turnover, and biomechanical load, they have served as important starting points for developmental and degenerative studies as well as preclinical studies for therapeutics. Although human patient studies are difficult to achieve in large sample sizes, the ability to compare human data to large sets of animal data allows investigators to more readily establish validity of and relevance within their findings.
The ideal outcome for future regenerative therapies is to be able to fully replace any damaged and diseased tissue with a substitute that has the capability to restore physiological function throughout the remainder of the patient’s lifetime. The best way to achieve this goal is to recapitulate the composition of the native tissue as closely as possible. Considering that organs and tissues in the body are in their healthiest state at the earliest points following completion of development and that the ultimate objective is to replicate native functional tissue, a key ingredient that is necessary to make significant progress towards building successful IVD regenerative strategies is a fully elucidated genetic and molecular profile of IVD development. Although multiple approaches to regenerative therapeutics have been under investigation for several years, we are still missing a comprehensive methodology that combines cellular gene expression, molecular signaling, and the ECM structure to construct native tissue-like replacements. Building on genetic and omics studies that have been completed thus far, in combination with multi-omics approaches, which allow for both temporal and spatial deconvolution of gene, protein, and other biomolecule expression at unprecedented resolution, we are well on our way to building a comprehensive profile of many aspects of the IVD. Taken together, these data will be crucial in furthering our understanding of development, maintenance, and pathology of the IVD, and will inform novel diagnostic and therapeutic advances in the future.
Author Contributions
TH, SR-K, and SP drafted and edited the manuscript.
Funding
This work was supported by funds from the Department of Medicine at Vanderbilt University Medical Center.
Conflict of Interest
The authors declare that the research was conducted in the absence of any commercial or financial relationships that could be construed as a potential conflict of interest.
Publisher’s Note
All claims expressed in this article are solely those of the authors and do not necessarily represent those of their affiliated organizations, or those of the publisher, the editors and the reviewers. Any product that may be evaluated in this article, or claim that may be made by its manufacturer, is not guaranteed or endorsed by the publisher.
Acknowledgments
The authors thank the Interdisciplinary Graduate Program in the Biological and Biomedical Sciences and the Quantitative and Chemical Biology Program at the Vanderbilt University School of Medicine. Figures were generated using BioRender.
Supplementary Material
The Supplementary Material for this article can be found online at: https://www.frontiersin.org/articles/10.3389/fcell.2022.841831/full#supplementary-material
References
Adams, M. A., and Hutton, W. C. (1986). The Effect of Posture on Diffusion into Lumbar Intervertebral Discs. J. Anat. 147, 121–134.
Adams, M. A., and Roughley, P. J. (2006). What Is Intervertebral Disc Degeneration, and what Causes it? Spine 31, 2151–2161. doi:10.1097/01.brs.0000231761.73859.2c
Adler, J. H., Schoenbaum, M., and Silberberg, R. (1983). Early Onset of Disk Degeneration and Spondylosis in Sand Rats (Psammomys Obesus). Vet. Pathol. 20, 13–22. doi:10.1177/030098588302000102
Alexander, D. I. (1986). Chemonucleolysis as Treatment for Herniated Lumbar Disc. Can. Fam. Physician 32, 114–117.
Alfred, J. B., Rance, K., Taylor, B. A., Phillips, S. J., Abbott, C. M., and Jackson, I. J. (1997). Mapping in the Region of Danforth's Short Tail and the Localization of Tail Length Modifiers. Genome Res. 7, 108–117. doi:10.1101/gr.7.2.108
Ali, R., Le Maitre, C., Richardson, S., Hoyland, J., and Freemont, A. (2008). Connective Tissue Growth Factor Expression in Human Intervertebral Disc: Implications for Angiogenesis in Intervertebral Disc Degeneration. Biotech. Histochem. 83, 239–245. doi:10.1080/10520290802539186
Alini, M., Eisenstein, S. M., Ito, K., Little, C., Kettler, A. A., Masuda, K., et al. (2008). Are Animal Models Useful for Studying Human Disc Disorders/degeneration? Eur. Spine J. 17, 2–19. doi:10.1007/s00586-007-0414-y
Alkhatib, B., Ban, G. I., Williams, S., and Serra, R. (2018). IVD Development: Nucleus Pulposus Development and Sclerotome Specification. Curr. Mol. Biol. Rep. 4 (3 4), 132–141. doi:10.1007/s40610-018-0100-3
Anderson, P. A., Rouleau, J. P., Bryan, V. E., and Carlson, C. S. (2003b). Wear Analysis of the Bryan Cervical Disc Prosthesis. Spine 28, S186–S194. doi:10.1097/01.brs.0000092212.42388.79
Ang, S.-L., and Rossant, J. (1994). HNF-3β Is Essential for Node and Notochord Formation in Mouse Development. Cell 78, 561–574. doi:10.1016/0092-8674(94)90522-3
Annunen, S., Paassilta, P., Lohiniva, J., Perälä, M., Pihlajamaa, T., Karppinen, J., et al. (1999). An Allele of COL9A2 Associated with Intervertebral Disc Disease. Science 285, 409–412. doi:10.1126/science.285.5426.409
Aslam, B., Basit, M., Nisar, M. A., Khurshid, M., and Rasool, M. H. (2017). Proteomics: Technologies and Their Applications. J. Chromatogr. Sci. 55, 182–196. doi:10.1093/chromsci/bmw167
Baffi, M. O., Slattery, E., Sohn, P., Moses, H. L., Chytil, A., and Serra, R. (2004). Conditional Deletion of the TGF-β Type II Receptor in Col2a Expressing Cells Results in Defects in the Axial Skeleton without Alterations in Chondrocyte Differentiation or Embryonic Development of Long Bones. Developmental Biol. 276, 124–142. doi:10.1016/j.ydbio.2004.08.027
Bagnat, M., and Gray, R. S. (2020). Development of a Straight Vertebrate Body axis. Development 147. doi:10.1242/dev.175794
Bailey, J. F., Fields, A. J., Liebenberg, E., Mattison, J. A., Lotz, J. C., and Kramer, P. A. (2014). Comparison of Vertebral and Intervertebral Disc Lesions in Aging Humans and Rhesus Monkeys. Osteoarthritis and Cartilage 22, 980–985. doi:10.1016/j.joca.2014.04.027
Barcellona, M. N., Speer, J. E., Fearing, B. V., Jing, L., Pathak, A., Gupta, M. C., et al. (2020). Control of Adhesive Ligand Density for Modulation of Nucleus Pulposus Cell Phenotype. Biomaterials 250, 120057. doi:10.1016/j.biomaterials.2020.120057
Barendse, G. A. M., Van Den Berg, S. G. M., Kessels, A. H. F., Weber, W. E. J., and Van Kleef, M. (2001). Randomized Controlled Trial of Percutaneous Intradiscal Radiofrequency Thermocoagulation for Chronic Discogenic Back Pain. Spine 26, 287–292. doi:10.1097/00007632-200102010-00014
Baumgartner, L., Wuertz-Kozak, K., Le Maitre, C. L., Wignall, F., Richardson, S. M., Hoyland, J., et al. (2021). Multiscale Regulation of the Intervertebral Disc: Achievements in Experimental, In Silico, and Regenerative Research. Int. J. Mol. Sci. 22. doi:10.3390/ijms22020703
Bedore, J., Sha, W., McCann, M. R., Liu, R., Leask, A., and Séguin, C. A. (2013). Impaired Intervertebral Disc Development And Premature Disc Degeneration In Mice With Notochord-Specific Deletion Of CCN2. Arthritis Rheum. 65, 2634–1644. doi:10.1002/art.38075
Behrens, A., Haigh, J., Mechta-Grigoriou, F., Nagy, A., Yaniv, M., and Wagner, E. F. (2003). Impaired Intervertebral Disc Formation In The Absence Of Jun. Development 130, 103–109. doi:10.1242/dev.00186
Bergknut, N., Rutges, J. P. H. J., Kranenburg, H.-J. C., Smolders, L. A., Hagman, R., Smidt, H.-J., et al. (2012). The Dog as an Animal Model for Intervertebral Disc Degeneration? Spine 37, 351–358. doi:10.1097/brs.0b013e31821e5665
Bi, W., Deng, J. M., Zhang, Z., Behringer, R. R., and De Crombrugghe, B. (1999). Sox9 Is Required for Cartilage Formation. Nat. Genet. 22 (1 22), 85–89. doi:10.1038/8792
Bibby, S. R. S., Jones, D. A., Ripley, R. M., and Urban, J. P. G. (2005). Metabolism of the Intervertebral Disc: Effects of Low Levels of Oxygen, Glucose, and pH on Rates of Energy Metabolism of Bovine Nucleus Pulposus Cells. Spine 30, 487–496. doi:10.1097/01.brs.0000154619.38122.47
Blanco, J. F., Villarón, E. M., Pescador, D., Da Casa, C., Gómez, V., Redondo, A. M., et al. (2019). Autologous Mesenchymal Stromal Cells Embedded in Tricalcium Phosphate for Posterolateral Spinal Fusion: Results of a Prospective Phase I/II Clinical Trial with Long-Term Follow-Up. Stem Cel Res Ther 10, 63. doi:10.1186/s13287-019-1166-4
Blanco, J. F., Graciani, I. F., Sanchez-Guijo, F. M., Muntión, S., Hernandez-Campo, P., Santamaria, C., et al. (2010). Isolation and Characterization of Mesenchymal Stromal Cells from Human Degenerated Nucleus Pulposus. Spine 35, 2259–2265. doi:10.1097/brs.0b013e3181cb8828
Boos, N., Weissbach, S., Rohrbach, H., Weiler, C., Spratt, K. F., and Nerlich, A. G. (2002). Classification of Age-Related Changes in Lumbar Intervertebral Discs. Spine 27, 2631–2644. doi:10.1097/00007632-200212010-00002
Borrelli, C., and Buckley, C. T. (2020). Injectable Disc-Derived ECM Hydrogel Functionalised with Chondroitin Sulfate for Intervertebral Disc Regeneration. Acta Biomater. 117, 142–155. doi:10.1016/j.actbio.2020.10.002
Bowles, R. D., and Setton, L. A. (2017). Biomaterials for Intervertebral Disc Regeneration and Repair. Biomaterials 129, 54–67. doi:10.1016/j.biomaterials.2017.03.013
Bradford, D. S., Cooper, K. M., and Oegema, T. R. (1983). Chymopapain, Chemonucleolysis, and Nucleus Pulposus Regeneration. J. Bone Jt. Surg. 65, 1220–1231. doi:10.2106/00004623-198365090-00002
Bridgen, D. T., Gilchrist, C. L., Richardson, W. J., Isaacs, R. E., Brown, C. R., Yang, K. L., et al. (2013). Integrin-mediated Interactions with Extracellular Matrix Proteins for Nucleus Pulposus Cells of the Human Intervertebral Disc. J. Orthop. Res. 31, 1661–1667. doi:10.1002/jor.22395
Buchbinder, R., Van Tulder, M., Öberg, B., Costa, L. M., Woolf, A., Schoene, M., et al. (2018). Low Back Pain: a Call for Action. The Lancet 391, 2384–2388. doi:10.1016/s0140-6736(18)30488-4
Buckwalter, J. A. (1995). Aging and Degeneration of the Human Intervertebral Disc. Spine 20, 1307–1314. doi:10.1097/00007632-199506000-00022
Caldeira, J., Santa, C., Osório, H., Molinos, M., Manadas, B., Gonçalves, R., et al. (2017). Matrisome Profiling during Intervertebral Disc Development and Ageing. Sci. Rep. 7, 11629. doi:10.1038/s41598-017-11960-0
Calió, M., Gantenbein, B., Egli, M., Poveda, L., and Ille, F. (2021). The Cellular Composition of Bovine Coccygeal Intervertebral Discs: A Comprehensive Single-Cell RNAseq Analysis. Int. J. Mol. Sci. 22. doi:10.3390/ijms22094917
Calvo-Echenique, A., Cegoñino, J., Correa-Martín, L., Bances, L., and Palomar, A. P.-d. (2018). Intervertebral Disc Degeneration: an Experimental and Numerical Study Using a Rabbit Model. Med. Biol. Eng. Comput. 56, 865–877. doi:10.1007/s11517-017-1738-3
Cassidy, J. J., Hiltner, A., and Baer, E. (1989). Hierarchical Structure of the Intervertebral Disc. Connect. Tissue Res. 23, 75–88. doi:10.3109/03008208909103905
Chang, G., Kim, H.-J., Kaplan, D., Vunjak-Novakovic, G., and Kandel, R. A. (2007). Porous Silk Scaffolds Can Be Used for Tissue Engineering Annulus Fibrosus. Eur. Spine J. 16, 1848–1857. doi:10.1007/s00586-007-0364-4
Chatterjee, S., Sivakamasundari, V., Yap, S. P., Kraus, P., Kumar, V., Xing, X., et al. (2014). In Vivo genome-wide Analysis of Multiple Tissues Identifies Gene Regulatory Networks, Novel Functions and Downstream Regulatory Genes for Bapx1 and its Co-regulation with Sox9 in the Mammalian Vertebral Column. BMC Genomics 15, 1072. doi:10.1186/1471-2164-15-1072
Chen, J., Jing, L., Gilchrist, C. L., Richardson, W. J., Fitch, R. D., and Setton, L. A. (2009). Expression of Laminin Isoforms, Receptors, and Binding Proteins Unique to Nucleus Pulposus Cells of Immature Intervertebral Disc. Connect. Tissue Res. 50, 294–306. doi:10.1080/03008200802714925
Chen, J., Lee, E. J., Jing, L., Christoforou, N., Leong, K. W., and Setton, L. A. (2013). Differentiation of Mouse Induced Pluripotent Stem Cells (iPSCs) into Nucleus Pulposus-like Cells In Vitro. PLOS ONE 8, e75548. doi:10.1371/journal.pone.0075548
Chen, P., Ning, L., Qiu, P., Mo, J., Mei, S., Xia, C., et al. (2019). Photo‐crosslinked Gelatin‐hyaluronic Acid Methacrylate Hydrogel‐committed Nucleus Pulposus‐like Differentiation of Adipose Stromal Cells for Intervertebral Disc Repair. J. Tissue Eng. Regen. Med. 13, 682–693. doi:10.1002/term.2841
Chen, W.-J., Tsai, T.-T., Chen, L.-H., Niu, C.-C., Lai, P.-L., Fu, T.-S., et al. (2005). The Fusion Rate of Calcium Sulfate with Local Autograft Bone Compared with Autologous Iliac Bone Graft for Instrumented Short-Segment Spinal Fusion. Spine 30, 2293–2297. doi:10.1097/01.brs.0000182087.35335.05
Chiba, K., Matsuyama, Y., Seo, T., and Toyama, Y. (2018). Condoliase for the Treatment of Lumbar Disc Herniation. Spine 43, E869–E876. doi:10.1097/brs.0000000000002528
Choi, H., Tessier, S., Silagi, E. S., Kyada, R., Yousefi, F., Pleshko, N., et al. (2018). A Novel Mouse Model of Intervertebral Disc Degeneration Shows Altered Cell Fate and Matrix Homeostasis. Matrix Biol. 70, 102–122. doi:10.1016/j.matbio.2018.03.019
Choi, K.-S., Cohn, M. J., and Harfe, B. D. (2008). Identification of Nucleus Pulposus Precursor Cells and Notochordal Remnants in the Mouse: Implications for Disk Degeneration and Chordoma Formation. Dev. Dyn. 237, 3953–3958. doi:10.1002/dvdy.21805
Choi, K.-S., and Harfe, B. D. (2011). Hedgehog Signaling Is Required for Formation of the Notochord Sheath and Patterning of Nuclei Pulposi within the Intervertebral Discs. Proc. Natl. Acad. Sci. 108, 9484–9489. doi:10.1073/pnas.1007566108
Choi, U. Y., Joshi, H. P., Payne, S., Kim, K. T., Kyung, J. W., Choi, H., et al. (2020). An Injectable Hyaluronan-Methylcellulose (HAMC) Hydrogel Combined with Wharton's Jelly-Derived Mesenchymal Stromal Cells (WJ-MSCs) Promotes Degenerative Disc Repair. Int. J. Mol. Sci. 21, 1–20. doi:10.3390/ijms21197391
Chou, R., Baisden, J., Carragee, E. J., Resnick, D. K., Shaffer, W. O., and Loeser, J. D. (2009). Surgery for Low Back Pain: A Review of the Evidence for an American Pain Society Clinical Practice Guideline. Spine 34, 1094–1109. doi:10.1097/brs.0b013e3181a105fc
Christ, B., Huang, R., and Scaal, M. (2007). Amniote Somite Derivatives. Dev. Dyn. 236, 2382–2396. doi:10.1002/dvdy.21189
Christ, B., and Wilting, J. (1992). From Somites to Vertebral Column. Ann. Anat. - Anatomischer Anzeiger 174, 23–32. doi:10.1016/s0940-9602(11)80337-7
Christiani, T. R., Baroncini, E., Stanzione, J., and Vernengo, A. J. (2019). In Vitro evaluation of 3D Printed Polycaprolactone Scaffolds with Angle-Ply Architecture for Annulus Fibrosus Tissue Engineering. Regenerative Biomater. 6, 175–184. doi:10.1093/rb/rbz011
Clarke, L. E., McConnell, J. C., Sherratt, M. J., Derby, B., Richardson, S. M., and Hoyland, J. A. (2014). Growth Differentiation Factor 6 and Transforming Growth Factor-Beta Differentially Mediate Mesenchymal Stem Cell Differentiation, Composition, and Micromechanical Properties of Nucleus Pulposus Constructs. Arthritis Res. Ther. 16, R67–R13. doi:10.1186/ar4505
Clouet, J., Fusellier, M., Camus, A., Le Visage, C., and Guicheux, J. (2019). Intervertebral Disc Regeneration: From Cell Therapy to the Development of Novel Bioinspired Endogenous Repair Strategies. Adv. Drug Deliv. Rev. 146, 306–324. doi:10.1016/j.addr.2018.04.017
Clouet, J., Grimandi, G., Pot-Vaucel, M., Masson, M., Fellah, H. B., Guigand, L., et al. (2009). Identification of Phenotypic Discriminating Markers for Intervertebral Disc Cells and Articular Chondrocytes. Rheumatology 48, 1447–1450. doi:10.1093/rheumatology/kep262
Colombier, P., Camus, A., Lescaudron, L., Clouet, J., and Guicheux, J. (2014a). Intervertebral Disc Regeneration: a Great challenge for Tissue Engineers. Trends Biotechnol. 32, 433–435. doi:10.1016/j.tibtech.2014.05.006
Colombier, P., Clouet, J., Boyer, C., Ruel, M., Bonin, G., Lesoeur, J., et al. (2016). TGF-β1 and GDF5 Act Synergistically to Drive the Differentiation of Human Adipose Stromal Cells toward Nucleus Pulposus-like Cells. STEM CELLS 34, 653–667. doi:10.1002/stem.2249
Colombier, P., Clouet, J., Hamel, O., Lescaudron, L., and Guicheux, J. (2014b). The Lumbar Intervertebral Disc: From Embryonic Development to Degeneration. Jt. Bone Spine 81, 125–129. doi:10.1016/j.jbspin.2013.07.012
Colombier, P., Halgand, B., Chédeville, C., Chariau, C., François-Campion, V., Kilens, S., et al. (2020). NOTO Transcription Factor Directs Human Induced Pluripotent Stem Cell-Derived Mesendoderm Progenitors to a Notochordal Fate. Cells 9. doi:10.3390/cells9020509
Comella, K., Silbert, R., and Parlo, M. (2017). Effects of the Intradiscal Implantation of Stromal Vascular Fraction Plus Platelet Rich Plasma in Patients with Degenerative Disc Disease. J. Transl Med. 15, 12. doi:10.1186/s12967-016-1109-0
Cunningham, B. W., Lowery, G. L., Serhan, H. A., Dmitriev, A. E., Orbegoso, C. M., McAfee, P. C., et al. (2002). Total Disc Replacement Arthroplasty Using the AcroFlex Lumbar Disc: a Non-human Primate Model. Eur. Spine J. 11, S115–S123. doi:10.1007/s00586-002-0481-z
De Geer, C. M. (2018). Intervertebral Disk Nutrients and Transport Mechanisms in Relation to Disk Degeneration: A Narrative Literature Review. J. Chiropractic Med. 17, 97–105. doi:10.1016/j.jcm.2017.11.006
De Souza, I. M. B., Sakaguchi, T. F., Yuan, S. L. K., Matsutani, L. A., do Espírito-Santo, A. S., Pereira, C. A. B., et al. (2019). Prevalence of Low Back Pain in the Elderly Population: a Systematic Review. Clinics (Sao Paulo) 74, e789. doi:10.6061/clinics/2019/e789
Dhar, D., and Hsi-En Ho, J. (2009). Stem Cell Research Policies Around the World. Yale J. Biol. Med. 82, 113–115.
Diamant, B., Karlsson, J., and Nachemson, A. (1968). Correlation between Lactate Levels and pH in Discs of Patients with Lumbar Rhizopathies. Experientia 24, 1195–1196. doi:10.1007/bf02146615
Diaz-Hernandez, M. E., Khan, N. M., Trochez, C. M., Yoon, T., Maye, P., Presciutti, S. M., et al. (2020). Derivation of Notochordal Cells from Human Embryonic Stem Cells Reveals Unique Regulatory Networks by Single Cell-Transcriptomics. J. Cel Physiol 235, 5241–5255. doi:10.1002/jcp.29411
Doench, I., Tran, T., David, L., Montembault, A., Viguier, E., Gorzelanny, C., et al. (2019). Cellulose Nanofiber-Reinforced Chitosan Hydrogel Composites for Intervertebral Disc Tissue Repair. Biomimetics 4, 19. doi:10.3390/biomimetics4010019
Dou, Y., Sun, X., Ma, X., Zhao, X., and Yang, Q. (2021). Intervertebral Disk Degeneration: The Microenvironment and Tissue Engineering Strategies. Front. Bioeng. Biotechnol. 9, 592118. doi:10.3389/fbioe.2021.592118
Du, L., Zhu, M., Yang, Q., Zhang, J., Ma, X., Kong, D., et al. (2014). A Novel Integrated Biphasic Silk Fibroin Scaffold for Intervertebral Disc Tissue Engineering. Mater. Lett. 117, 237–240. doi:10.1016/j.matlet.2013.12.029
Du, Y., Wang, Z., Wu, Y., Liu, C., and Zhang, L. (2021). Intervertebral Disc Stem/Progenitor Cells: A Promising "Seed" for Intervertebral Disc Regeneration. Stem Cell Int 2021, 2130727. doi:10.1155/2021/2130727
Elmasry, S., Asfour, S., De Rivero Vaccari, J. P., and Travascio, F. (2015). Effects of Tobacco Smoking on the Degeneration of the Intervertebral Disc: A Finite Element Study. PLoS ONE 10, e0136137. doi:10.1371/journal.pone.0136137
Eyre, D. R., Caterson, B., Benya, P., Heingard, D., Oegema, T. R., Pearce, R. H., et al. (1991). “The Intervertebral Disc,” in New Perspectives on Low Back Pain. Editor F. J. Gordon S (Philadelphia, PA: American Institute of Orthopaedic Surgeons), 147–209.
Feng, C., Yang, M., Lan, M., Liu, C., Zhang, Y., Huang, B., et al. (2017a). ROS: Crucial Intermediators in the Pathogenesis of Intervertebral Disc Degeneration. Oxidative Med. Cell Longevity 2017.
Feng, G., Zhang, Z., Dang, M., Rambhia, K. J., and Ma, P. X. (2020). Nanofibrous Spongy Microspheres to Deliver Rabbit Mesenchymal Stem Cells and Anti-miR-199a to Regenerate Nucleus Pulposus and Prevent Calcification. Biomaterials 256, 120213. doi:10.1016/j.biomaterials.2020.120213
Feng, G., Zhang, Z., Dang, M., Zhang, X., Doleyres, Y., Song, Y., et al. (2017b). Injectable Nanofibrous Spongy Microspheres for NR4A1 Plasmid DNA Transfection to Reverse Fibrotic Degeneration and Support Disc Regeneration. Biomaterials 131, 86–97. doi:10.1016/j.biomaterials.2017.03.029
Feng, Y., Egan, B., and Wang, J. (2016). Genetic Factors in Intervertebral Disc Degeneration. Genes Dis. 3, 178–185. doi:10.1016/j.gendis.2016.04.005
Fernandes, L. M., Khan, N. M., Trochez, C. M., Duan, M., Diaz-Hernandez, M. E., Presciutti, S. M., et al. (2020). Single-cell RNA-Seq Identifies Unique Transcriptional Landscapes of Human Nucleus Pulposus and Annulus Fibrosus Cells. Sci. Rep. 10, 15263. doi:10.1038/s41598-020-72261-7
Ferrara, L. A. (2012). The Biomechanics of Cervical Spondylosis. Adv. Orthop. 2012, 493605. doi:10.1155/2012/493605
Fogelholm, R. R., and Alho, A. V. A. (2001). Smoking and Intervertebral Disc Degeneration. Med. hypotheses 56, 537–539. doi:10.1054/mehy.2000.1253
Foster, J. W., Dominguez-Steglich, M. A., Guioli, S., Kwok, C., Weller, P. A., Stevanović, M., et al. (1994). Campomelic Dysplasia and Autosomal Sex Reversal Caused by Mutations in an SRY-Related Gene. Nature 372, 525–530. doi:10.1038/372525a0
Fouquet, B., Weinstein, B. M., Serluca, F. C., and Fishman, M. C. (1997). Vessel Patterning in the Embryo of the Zebrafish: Guidance by Notochord. Developmental Biol. 183, 37–48. doi:10.1006/dbio.1996.8495
Francisco, A. T., Mancino, R. J., Bowles, R. D., Brunger, J. M., Tainter, D. M., Chen, Y.-T., et al. (2013). Injectable Laminin-Functionalized Hydrogel for Nucleus Pulposus Regeneration. Biomaterials 34, 7381–7388. doi:10.1016/j.biomaterials.2013.06.038
Freeman, B. J. C., Fraser, R. D., Cain, C. M. J., Hall, D. J., and Chapple, D. C. L. (2005). A Randomized, Double-Blind, Controlled Trial. Spine 30, 2369–2377. doi:10.1097/01.brs.0000186587.43373.f2
Freidin, M. B., Tsepilov, Y. A., Palmer, M., Karssen, L. C., Suri, P., Aulchenko, Y. S., et al. (2019). Insight into the Genetic Architecture of Back Pain and its Risk Factors from a Study of 509,000 Individuals. Pain 160, 1361–1373. doi:10.1097/j.pain.0000000000001514
Furukawa, T., Ito, K., Nuka, S., Hashimoto, J., Takei, H., Takahara, M., et al. (2009). Absence Of Biglycan Accelerates The Degenerative Process In Mouse Intervertebral Disc. Spine 34 (25), E911–E917. doi:10.1097/BRS.0b013e3181b7c7ec
Gal, J. (2002). Mammalian Spinal Biomechanics: Postural Support in Seated Macaques. J. Exp. Biol. 205, 1703–1707. doi:10.1242/jeb.205.12.1703
Gan, Y., He, J., Zhu, J., Xu, Z., Wang, Z., Yan, J., et al. (2021). Spatially Defined Single-Cell Transcriptional Profiling Characterizes Diverse Chondrocyte Subtypes and Nucleus Pulposus Progenitors in Human Intervertebral Discs. Bone Res. 9 (1 9), 37–15. doi:10.1038/s41413-021-00163-z
Gan, Y., Li, P., Wang, L., Mo, X., Song, L., Xu, Y., et al. (2017). An Interpenetrating Network-Strengthened and Toughened Hydrogel that Supports Cell-Based Nucleus Pulposus Regeneration. Biomaterials 136, 12–28. doi:10.1016/j.biomaterials.2017.05.017
Gantenbein-Ritter, B., Benneker, L. M., Alini, M., and Grad, S. (2011). Differential Response of Human Bone Marrow Stromal Cells to Either TGF-Β1 or rhGDF-5. Eur. Spine J. 20, 962–971. doi:10.1007/s00586-010-1619-z
Gao, F., Mao, T., Sun, W., Guo, W., Wang, Y., Li, Z., et al. (2015). An Updated Meta-Analysis Comparing Artificial Cervical Disc Arthroplasty (CDA) versus Anterior Cervical Discectomy and Fusion (ACDF) for the Treatment of Cervical Degenerative Disc Disease (CDDD). Spine 40, 1816–1823. doi:10.1097/brs.0000000000001138
García-Cosamalón, J., Del Valle, M. E., Calavia, M. G., García-Suárez, O., López-Muñiz, A., Otero, J., et al. (2010). Intervertebral Disc, Sensory Nerves and Neurotrophins: Who Is Who in Discogenic Pain? J. Anat. 217, 1–15.
Gelalis, I., Gkiatas, I., Spiliotis, A., Papadopoulos, D., Pakos, E., Vekris, M., et al. (2019). Current Concepts in Intradiscal Percutaneous Minimally Invasive Procedures for Chronic Low Back Pain. Asian J. Neurosurg. 14, 657–669. doi:10.4103/ajns.AJNS_119_17
Ghiselli, G., Wang, J. C., Bhatia, N. N., Hsu, W. K., and Dawson, E. G. (2004). Adjacent Segment Degeneration in the Lumbar Spine. The J. Bone Jt. Surgery-American Volume 86, 1497–1503. doi:10.2106/00004623-200407000-00020
Gilson, A., Dreger, M., and Urban, J. P. (2010). Differential Expression Level of Cytokeratin 8 in Cells of the Bovine Nucleus Pulposus Complicates the Search for Specific Intervertebral Disc Cell Markers. Arthritis Res. Ther. 12, R24–R12. doi:10.1186/ar2931
Girardi, F. P., and Cammisa, F. P. (2003). The Effect of Bone Graft Extenders to Enhance the Performance of Iliac Crest Bone Grafts in Instrumented Lumbar Spine Fusion. Orthopedics 26, s545–8. doi:10.3928/0147-7447-20030502-03
Gorth, D. J., Shapiro, I. M., and Risbud, M. V. (2019). A New Understanding of the Role of IL-1 in Age-Related Intervertebral Disc Degeneration in a Murine Model. J. Bone Miner. Res. 34, 1531–1542. doi:10.1002/jbmr.3714
Greg Anderson, D., Li, X., Tannoury, T., Beck, G., and Balian, G. (2003). A Fibronectin Fragment Stimulates Intervertebral Disc Degeneration In Vivo. Spine 28, 2338–2345. doi:10.1097/01.brs.0000096943.27853.bc
Gruber, H. E., and Hanley, E. N. (1998). Analysis of Aging and Degeneration of the Human Intervertebral Disc. Spine 23, 751–757. doi:10.1097/00007632-199804010-00001
Grunhagen, T., Wilde, G., Soukane, D. M., Shirazi-Adl, S. A., and Urban, J. P. G. (2006). Nutrient Supply and Intervertebral Disc Metabolism. J. Bone Jt. Surg. 88, 30–35. doi:10.2106/jbjs.e.01290
Gullbrand, S. E., Malhotra, N. R., Schaer, T. P., Zawacki, Z., Martin, J. T., Bendigo, J. R., et al. (2017a). A Large Animal Model that Recapitulates the Spectrum of Human Intervertebral Disc Degeneration. Osteoarthritis and cartilage 25, 146–156. doi:10.1016/j.joca.2016.08.006
Gullbrand, S. E., Schaer, T. P., Agarwal, P., Bendigo, J. R., Dodge, G. R., Chen, W., et al. (2017b). Translation of an Injectable Triple-Interpenetrating-Network Hydrogel for Intervertebral Disc Regeneration in a Goat Model. Acta Biomater. 60, 201–209. doi:10.1016/j.actbio.2017.07.025
Guo, P., Yuan, Y., and Chi, F. (2014). Biomimetic Alginate/polyacrylamide Porous Scaffold Supports Human Mesenchymal Stem Cell Proliferation and Chondrogenesis. Mater. Sci. Eng. C 42, 622–628. doi:10.1016/j.msec.2014.06.013
Guo, W., Douma, L., Hu, M. H., Eglin, D., Alini, M., Šećerović, A., et al. (2022). Hyaluronic Acid-Based Interpenetrating Network Hydrogel as a Cell Carrier for Nucleus Pulposus Repair. Carbohydr. Polym. 277, 118828. doi:10.1016/j.carbpol.2021.118828
Gupta, A., Kukkar, N., Sharif, K., Main, B. J., Albers, C. E., and Iii, S. F. E.-A. (2015). Bone Graft Substitutes for Spine Fusion: A Brief Review. Wjo 6, 449–456. doi:10.5312/wjo.v6.i6.449
Hangai, M., Kaneoka, K., Kuno, S., Hinotsu, S., Sakane, M., Mamizuka, N., et al. (2008). Factors Associated with Lumbar Intervertebral Disc Degeneration in the Elderly. Spine J. 8, 732–740. doi:10.1016/j.spinee.2007.07.392
Harfe, B. D., Scherz, P. J., Nissim, S., Tian, H., Mcmahon, A. P., and Tabin, C. J. (2004). Evidence for an Expansion-Based Temporal Shh Gradient in Specifying Vertebrate Digit Identities. Cell 118, 517–528. doi:10.1016/j.cell.2004.07.024
Hayes, A. J., Benjamin, M., and Ralphs, J. R. (1999). Role of Actin Stress Fibres in the Development of the Intervertebral Disc: Cytoskeletal Control of Extracellular Matrix Assembly. Dev. Dyn. 215, 179–189. doi:10.1002/(sici)1097-0177(199907)215:3<179::aid-aja1>3.0.co;2-q
Heller, J. G., Sasso, R. C., Papadopoulos, S. M., Anderson, P. A., Fessler, R. G., Hacker, R. J., et al. (2009). Comparison of BRYAN Cervical Disc Arthroplasty with Anterior Cervical Decompression and Fusion. Spine 34, 101–107. doi:10.1097/brs.0b013e31818ee263
Henriksson, H. B., Svanvik, T., Jonsson, M., Hagman, M., Horn, M., Lindahl, A., et al. (2009). Transplantation of Human Mesenchymal Stems Cells into Intervertebral Discs in a Xenogeneic Porcine Model. Spine 34, 141–148. doi:10.1097/brs.0b013e31818f8c20
Higuchi, M., Abe, K., and Kaneda, K. (1983). Changes in the Nucleus Pulposus of the Intervertebral Disc in Bipedal Mice. Clin. Orthopaedics Relat. Res. 175, 251–257. doi:10.1097/00003086-198305000-00042
Hiyama, A., Mochida, J., Iwashina, T., Omi, H., Watanabe, T., Serigano, K., et al. (2008). Transplantation of Mesenchymal Stem Cells in a Canine Disc Degeneration Model. J. Orthop. Res. 26, 589–600. doi:10.1002/jor.20584
Hollander, A. P., Heathfield, T. F., Liu, J. J., Pidoux, I., Roughley, P. J., Mort, J. S., et al. (1996). Enhanced Denaturation of the ?1(II) Chains of Type-II Collagen in normal Adult Human Intervertebral Discs Compared with Femoral Articular Cartilage. J. Orthop. Res. 14, 61–66. doi:10.1002/jor.1100140111
Holm, S., Holm, A. K., Ekström, L., Karladani, A., and Hansson, T. (2004). Experimental Disc Degeneration Due to Endplate Injury. J. Spinal Disord. Tech. 17, 64–71. doi:10.1097/00024720-200402000-00012
Holm, S., Maroudas, A., Urban, J. P. G., Selstam, G., and Nachemson, A. (1981). Nutrition of the Intervertebral Disc: Solute Transport and Metabolism. Connect. Tissue Res. 8, 101–119. doi:10.3109/03008208109152130
Holm, S., and Nachemson, A. (1988). Nutrition of the Intervertebral Disc: Acute Effects of Cigarette Smoking: An Experimental Animal Study. Upsala J. Med. Sci. 93, 91–99. doi:10.1517/03009734000000042
Hoy, D., March, L., Brooks, P., Blyth, F., Woolf, A., Bain, C., et al. (2014). The Global burden of Low Back Pain: Estimates from the Global Burden of Disease 2010 Study. Ann. Rheum. Dis. 73, 968–974. doi:10.1136/annrheumdis-2013-204428
Hu, J., Chen, B., Guo, F., Du, J., Gu, P., Lin, X., et al. (2012). Injectable Silk Fibroin/polyurethane Composite Hydrogel for Nucleus Pulposus Replacement. J. Mater. Sci. Mater. Med. 23, 711–722. doi:10.1007/s10856-011-4533-y
Huang, Y.-C., Urban, J. P. G., and Luk, K. D. K. (2014). Intervertebral Disc Regeneration: Do Nutrients lead the Way? Nat. Rev. Rheumatol. 10, 561–566. doi:10.1038/nrrheum.2014.91
Humzah, M. D., and Soames, R. W. (1988). Human Intervertebral Disc: Structure and Function. Anat. Rec. 220, 337–356. doi:10.1002/ar.1092200402
Hunter, C. J., Matyas, J. R., and Duncan, N. A. (2003). The Three-Dimensional Architecture of the Notochordal Nucleus Pulposus: Novel Observations on Cell Structures in the Canine Intervertebral Disc. J. Anat. 202, 279–291. doi:10.1046/j.1469-7580.2003.00162.x
Hwang, P. Y., Chen, J., Jing, L., Hoffman, B. D., and Setton, L. A. (2014). The Role of Extracellular Matrix Elasticity and Composition in Regulating the Nucleus Pulposus Cell Phenotype in the Intervertebral Disc: A Narrative Review. J. Biomech. Eng. 136, 021010. doi:10.1115/1.4026360
Hynes, K., Menicanin, D., Mrozik, K., Gronthos, S., and Bartold, P. M. (2014). Generation of Functional Mesenchymal Stem Cells from Different Induced Pluripotent Stem Cell Lines. Stem Cell Development 23, 1084–1096. doi:10.1089/scd.2013.0111
Hynes, R. O., and Naba, A. (2012). Overview of the Matrisome—An Inventory of Extracellular Matrix Constituents and Functions. Cold Spring Harbor Perspect. Biol. 4. doi:10.1101/cshperspect.a004903
Iozzo, R. V., and Schaefer, L. (2015). Proteoglycan Form and Function: A Comprehensive Nomenclature of Proteoglycans. Matrix Biol. 42, 11–55. doi:10.1016/j.matbio.2015.02.003
Ishihara, H., and Urban, J. P. G. (1999). Effects of Low Oxygen Concentrations and Metabolic Inhibitors on Proteoglycan and Protein Synthesis Rates in the Intervertebral Disc. J. Orthop. Res. 17, 829–835. doi:10.1002/jor.1100170607
Iwahashi, M., Matsuzaki, H., Tokuhashi, Y., Wakabayashi, K., and Uematsu, Y. (2002). Mechanism of Intervertebral Disc Degeneration Caused by Nicotine in Rabbits to Explicate Intervertebral Disc Disorders Caused by Smoking. Spine 27, 1396–1401. doi:10.1097/00007632-200207010-00005
Janeczko, Ł., Janeczko, M., Chrzanowski, R., and Zieliński, G. (2014). The Role of Polymorphisms of Genes Encoding Collagen IX and XI in Lumbar Disc Disease. Neurologia i Neurochirurgia Polska 48, 60–62. doi:10.1016/j.pjnns.2013.04.001
Jin, H., Shen, J., Wang, B., Wang, M., Shu, B., and Chen, D. (2011). TGF-β Signaling Plays an Essential Role in the Growth and Maintenance of Intervertebral Disc Tissue. FEBS Lett. 585, 1209–1215. doi:10.1016/j.febslet.2011.03.034
Johnson, W. E. B., Eisenstein, S. M., and Roberts, S. (2001). Cell Cluster Formation in Degenerate Lumbar Intervertebral Discs Is Associated with Increased Disc Cell Proliferation. Connect. Tissue Res. 42, 197–207. doi:10.3109/03008200109005650
Kapural, L., and Houra, K. (2012). Annuloplasty Procedures for Chronic Discogenic Back Pain. Tech. Reg. Anesth. Pain Management 16, 95–101. doi:10.1053/j.trap.2013.02.004
Kapural, L., and Mekhail, N. (2007). Novel Intradiscal Biacuplasty (IDB) for the Treatment of Lumbar Discogenic Pain. Pain Pract. 7, 130–134. doi:10.1111/j.1533-2500.2007.00120.x
Katta, J. K., Marcolongo, M., Lowman, A., and Mansmann, K. A. (2007). Friction and Wear Behavior of Poly(vinyl Alcohol)/poly(vinyl Pyrrolidone) Hydrogels for Articular Cartilage Replacement. J. Biomed. Mater. Res. 83A, 471–479. doi:10.1002/jbm.a.31238
Katz, J. N. (2006). Lumbar Disc Disorders and Low-Back Pain. J. Bone Jt. Surgery-American Volume 88 (Suppl. 2), 21–24. doi:10.2106/00004623-200604002-00005
Kawaguchi, Y., Osada, R., Kanamori, M., Ishihara, H., Ohmori, K., Matsui, H., et al. (1999). Association between an Aggrecan Gene Polymorphism and Lumbar Disc Degeneration. Spine 24, 2456–2460. doi:10.1097/00007632-199912010-00006
Kepler, C. K., Anderson, G. D., Tannoury, C., and Ponnappan, R. K. (2011). Intervertebral Disk Degeneration and Emerging Biologic Treatments. Am. Acad. Orthopaedic Surgeon 19, 543–553. doi:10.5435/00124635-201109000-00005
Kimura, T., Nakata, K., Tsumaki, N., Miyamoto, S., Matsui, Y., Ebara, S., et al. (1996). Progressive Degeneration Of Articular Cartilage And Intervertebral Discs. An Experimental Study In Transgenic Mice Bearing A Type IX Collagen Mutation. Int. Orthop. 20, 177–181. doi:10.1007/s002640050058
Kondo, N., Yuasa, T., Shimono, K., Tung, W., Okabe, T., Yasuhara, R., et al. (2011). Intervertebral Disc Development Is Regulated by Wnt/β-Catenin Signaling. Spine 36, E513–E518. doi:10.1097/brs.0b013e3181f52cb5
Kong, C.-B., Jeon, D.-W., Chang, B.-S., Lee, J. H., Suk, K.-S., and Park, J.-B. (2010). Outcome of Spinal Fusion for Lumbar Degenerative Disease. Spine 35, 1489–1494. doi:10.1097/brs.0b013e3181c49fd0
Korecki, C. L., Costi, J. J., and Iatridis, J. C. (2008). Needle Puncture Injury Affects Intervertebral Disc Mechanics and Biology in an Organ Culture Model. Spine 33, 235–241. doi:10.1097/brs.0b013e3181624504
Kraus, P., Li, K., Sipes, D., Varden, L., Yerden, R., Henderson, A., et al. (2019). “Single-Cell Phenotyping of Complex Heterogeneous Tissue,” in Handbook of Single Cell Technologies. Editors T. S. Santra, and F.-G. Tseng (Singapore: Springer Singapore), 1–17. doi:10.1007/978-981-10-4857-9_16-1
Kroeber, M. W., Unglaub, F., Wang, H., Schmid, C., Thomsen, M., Nerlich, A., et al. (2002). New In Vivo Animal Model to Create Intervertebral Disc Degeneration and to Investigate the Effects of Therapeutic Strategies to Stimulate Disc Regeneration. Spine 27, 2684–2690. doi:10.1097/00007632-200212010-00007
Kudelko, M., Chen, P., Tam, V., Zhang, Y., Kong, O.-Y., Sharma, R., et al. (2021). PRIMUS: Comprehensive Proteomics of Mouse Intervertebral Discs that Inform Novel Biology and Relevance to Human Disease Modelling. Matrix Biol. Plus 12, 100082. doi:10.1016/j.mbplus.2021.100082
Kumaresan, S., Yoganandan, N., Pintar, F. A., Macias, M., and Cusick, J. F. (2003). Morphology of Young and Old Cervical Spine Intervertebral Disc Tissues. Biomed. Sci. Instrumentation, 141–146.
Lai, A., Moon, A., Purmessur, D., Skovrlj, B., Winkelstein, B. A., Cho, S. K., et al. (2015). Assessment of Functional and Behavioral Changes Sensitive to Painful Disc Degeneration. J. Orthop. Res. 33, 755–764. doi:10.1002/jor.22833
Lane, P. W., and Birkenmeier, C. S. (1993). Urogenital Syndrome (Us): a Developmental Mutation on Chromosome 2 of the Mouse. Mamm. Genome 4, 481–484. doi:10.1007/bf00364781
Lauerman, W. C., Platenberg, R. C., Cain, J. E., and Deeney, V. F. X. (1992). Age-Related Disk Degeneration. J. Spinal Disord. 5, 170–174. doi:10.1097/00002517-199206000-00004
Lefebvre, V. (2019). Roles And Regulation Of SOX Transcription Factors In Skeletogenesis. Curr. Top. Dev. Biol. 133, 171–193. doi:10.1016/bs.ctdb.2019.01.007
Le Maitre, C. L., Pockert, A., Buttle, D. J., Freemont, A. J., and Hoyland, J. A. (2007). Matrix Synthesis and Degradation in Human Intervertebral Disc Degeneration. Biochem. Soc. Trans. 35, 652–655. doi:10.1042/BST0350652
Leung, V. Y. L., Aladin, D. M. K., Lv, F., Tam, V., Sun, Y., Lau, R. Y. C., et al. (2014). Mesenchymal Stem Cells Reduce Intervertebral Disc Fibrosis and Facilitate Repair. Stem Cells 32, 2164–2177. doi:10.1002/stem.1717
Li, C., Bai, Q., Lai, Y., Tian, J., Li, J., Sun, X., et al. (2021). Advances and Prospects in Biomaterials for Intervertebral Disk Regeneration. Front. Bioeng. Biotechnol. 9. doi:10.3389/fbioe.2021.766087
Li, K., Kapper, D., Youngs, B., Kocsis, V., Mondal, S., Kraus, P., et al. (2019). Potential Biomarkers of the Mature Intervertebral Disc Identified at the Single Cell Level. J. Anat. 234, 16–32. doi:10.1111/joa.12904
Li, N., Li, Z., Li, R., Tian, J., Sun, G., Li, L., et al. (2017). A Novel Biomimetic Scaffold with hUCMSCs for Lumbar Fusion. J. Mater. Chem. B 5, 5996–6007. doi:10.1039/c6tb02640k
Li, S., Duance, V. C., and Blain, E. J. (2008). Zonal Variations in Cytoskeletal Element Organization, mRNA and Protein Expression in the Intervertebral Disc. J. Anat. 213, 725–732. doi:10.1111/j.1469-7580.2008.00998.x
Li, X., Leo, B. M., Beck, G., Balian, G., and Anderson, G. D. (2004). Collagen And Proteoglycan Abnormalities In The GDF-5-Deficient Mice and Molecular Changes When Treating Disk Cells With Recombinant Growth Factor. Spine 29, 2229–2234. doi:10.1097/01.brs.0000142427.82605.fb
Li, X., Yang, S., Han, L., Mao, K., and Yang, S. (2020). Ciliary IFT80 Is Essential For Intervertebral Disc Development And Maintenance. FASEB J. 34 (5), 6741–6756. doi:10.1096/fj.201902838R
Li, Z., Lang, G., Chen, X., Sacks, H., Mantzur, C., Tropp, U., et al. (2016). Polyurethane Scaffold with In Situ Swelling Capacity for Nucleus Pulposus Replacement. Biomaterials 84, 196–209. doi:10.1016/j.biomaterials.2016.01.040
Liang, C. Z., Li, H., Tao, Y. Q., Zhou, X. P., Yang, Z. R., Xiao, Y. X., et al. (2012). Dual Delivery for Stem Cell Differentiation Using Dexamethasone and bFGF In/on Polymeric Microspheres as a Cell Carrier for Nucleus Pulposus Regeneration. J. Mater. Sci. Mater. Med. 23, 1097–1107. doi:10.1007/s10856-012-4563-0
Liao, L., Jiang, H., Fan, Y., Lu, R. S., Wei, C., Takarada, T., et al. (2019). Runx2 is Required For Postnatal Intervertebral Disc Tissue Growth And Development. J. Cell. Physiol. 234 (5), 6679–6687. doi:10.1002/jcp.27410
Lie, M. U., Pedersen, L. M., Heuch, I., Winsvold, B., Gjerstad, J., Hasvik, E., et al. (2022). Low Back Pain with Persistent Radiculopathy; the Clinical Role of Genetic Variants in the Genes SOX5, CCDC26/GSDMC and DCC. Front. Genet. 12. doi:10.3389/fgene.2021.757632
Lipson, S. J., and Muir, H. (1981). Experimental Intervertebral Disc Degeneration. Morphologic and Proteoglycan Changes over Time. Arthritis Rheum. 24, 12–21. doi:10.1002/art.1780240103
Liu, C., Xiao, L., Zhang, Y., Zhao, Q., and Xu, H. (2020). Regeneration of Annulus Fibrosus Tissue Using a DAFM/PECUU-blended Electrospun Scaffold. J. Biomater. Sci. Polym. Edition 31, 2347–2361. doi:10.1080/09205063.2020.1812038
Liu, Y., Li, J. M., and Hu, Y. G. (2011). Transplantation of Gene-Modified Nucleus Pulposus Cells Reverses Rabbit Intervertebral Disc Degeneration. Chin. Med. J. (Engl) 124, 2431–2437.
Liu, Y., Fu, S., Rahaman, M. N., Mao, J. J., and Bal, B. S. (2015). Native Nucleus Pulposus Tissue Matrix Promotes Notochordal Differentiation of Human Induced Pluripotent Stem Cells with Potential for Treating Intervertebral Disc Degeneration. J. Biomed. Mater. Res. 103, 1053–1059. doi:10.1002/jbm.a.35243
Liu, Y., Rahaman, M. N., and Bal, B. S. (2014). Modulating Notochordal Differentiation of Human Induced Pluripotent Stem Cells Using Natural Nucleus Pulposus Tissue Matrix. PLOS ONE 9, e100885. doi:10.1371/journal.pone.0100885
Luk, K. D. K., Ruan, D. K., Chow, D. H. K., and Leong, J. C. Y. (1997). Intervertebral Disc Autografting in a Bipedal Animal Model. Clin. orthopaedics Relat. Res. 337, 13–26. doi:10.1097/00003086-199704000-00003
Luk, K. D. K., Ruan, D. K., Lu, D. S., and Fei, Z. Q. (2003). Fresh Frozen Intervertebral Disc Allografting in a Bipedal Animal Model. Spine 28, 864–869. doi:10.1097/01.brs.0000058710.01729.29
Lundon, K., and Bolton, K. (2001). Structure and Function of the Lumbar Intervertebral Disk in Health, Aging, and Pathologic Conditions. J. Orthop. Sports Phys. Ther. 31, 291–306. doi:10.2519/jospt.2001.31.6.291
Maeda, Y., Nakamura, E., Nguyen, M.-T., Suva, L. J., Swain, F. L., Razzaque, M. S., et al. (2007). Indian Hedgehog Produced by Postnatal Chondrocytes Is Essential for Maintaining a Growth Plate and Trabecular Bone. Proc. Natl. Acad. Sci. 104, 6382–6387. doi:10.1073/pnas.0608449104
Magnier, C., Boiron, O., Wendling-Mansuy, S., Chabrand, P., and Deplano, V. (2009). Nutrient Distribution and Metabolism in the Intervertebral Disc in the Unloaded State: A Parametric Study. J. Biomech. 42, 100–108. doi:10.1016/j.jbiomech.2008.10.034
Maier, J. A., Lo, Y., and Harfe, B. D. (2013). Foxa1 and Foxa2 Are Required for Formation of the Intervertebral Discs. PLoS ONE 8, e55528. doi:10.1371/journal.pone.0055528
Manchikanti, L., Singh, V., Calodney, A. K., Helm, S., Deer, T. R., Benyamin, R. M., et al. (2013). Percutaneous Lumbar Mechanical Disc Decompression Utilizing Dekompressor®: an Update of Current Evidence. Pain Physician 16, Se1–24. doi:10.36076/ppj.2013/16/se1
Maroudas, A. (1975). Biophysical Chemistry of Cartilaginous Tissues with Special Reference to Solute and Fluid Transport1. Bir 12, 233–248. doi:10.3233/bir-1975-123-416
Martin, J. T., Gorth, D. J., Beattie, E. E., Harfe, B. D., Smith, L. J., and Elliott, D. M. (2013). Needle Puncture Injury Causes Acute and Long-Term Mechanical Deficiency in a Mouse Model of Intervertebral Disc Degeneration. J. Orthop. Res. 31, 1276–1282. doi:10.1002/jor.22355
Masala, S., Salimei, F., Lacchè, A., Marcia, S., and Massari, F. (2019). Overview on Percutaneous Therapies of Disc Diseases. Medicina (Kaunas) 55. doi:10.3390/medicina55080471
Matsuyama, Y., and Chiba, K. (2019). Condoliase for Treatment of Lumbar Disc Herniation. Drugs Today 55, 17–23. doi:10.1358/dot.2019.55.1.2899445
Mauth, C., Bono, E., Bono, E., Haa, S., Paesold, G., Wiese, H., et al. (2009). Cell-seeded Polyurethane-Fibrin Structures - A Possible System for Intervertebral Disc Regeneration. eCM 18, 27–39. doi:10.22203/ecm.v018a03
McAfee, P. C., Cunningham, B. W., Orbegoso, C. M., Sefter, J. C., Dmitriev, A. E., and Fedder, I. L. (2003). Analysis of Porous Ingrowth in Intervertebral Disc Prostheses. Spine 28, 332–340. doi:10.1097/01.brs.0000048504.08086.42
McCann, M. R., Patel, P., Frimpong, A., Xiao, Y., Siqueira, W. L., and Séguin, C. A. (2015). Proteomic Signature of the Murine Intervertebral Disc. PLoS ONE 10, e0117807. doi:10.1371/journal.pone.0117807
McCann, M. R., Tamplin, O. J., Rossant, J., and Séguin, C. A. (2012). Tracing Notochord-Derived Cells Using a Noto-Cre Mouse: Implications for Intervertebral Disc Development. DMM Dis. Models Mech. 5, 73–82. doi:10.1242/dmm.008128
McGarry, T., Biniecka, M., Veale, D. J., and Fearon, U. (2018). Hypoxia, Oxidative Stress and Inflammation. Free Radic. Biol. Med. 125, 15–24. doi:10.1016/j.freeradbiomed.2018.03.042
McMillan, J. S., Jones, K., Forgan, L., Busija, L., Carey, R. P. L., De Silva, A. M., et al. (2021). Lumbar Spinal Fusion Surgery Outcomes in a Cohort of Injured Workers in the Victorian Workers' Compensation System. ANZ J. Surg. doi:10.1111/ans.17391
Meisel, H.-J., Agarwal, N., Hsieh, P. C., Skelly, A., Park, J.-B., Brodke, D., et al. (2019). Cell Therapy for Treatment of Intervertebral Disc Degeneration: A Systematic Review. Glob. Spine J. 9, 39S–52S. doi:10.1177/2192568219829024
Melrose, J., Ghosh, P., and Taylor, T. K. F. (2001). A Comparative Analysis of the Differential Spatial and Temporal Distributions of the Large (Aggrecan, Versican) and Small (Decorin, Biglycan, Fibromodulin) Proteoglycans of the Intervertebral Disc. J. Anat. 198, 3–15. doi:10.1046/j.1469-7580.2001.19810003.x
Meng, X., Zhuang, L., Wang, J., Liu, Z., Wang, Y., Xiao, D., et al. (2018). Hypoxia-Inducible Factor (HIF)-1alpha Knockout Accelerates Intervertebral Disc Degeneration In Mice. Int. J. Clin. Exp. Pathol. 11, 548–557.
Michalek, A. J., Funabashi, K. L., and Iatridis, J. C. (2010). Needle Puncture Injury of the Rat Intervertebral Disc Affects Torsional and Compressive Biomechanics Differently. Eur. Spine J. 19, 2110–2116. doi:10.1007/s00586-010-1473-z
Minogue, B. M., Richardson, S. M., Zeef, L. A. H., Freemont, A. J., and Hoyland, J. A. (2010). Characterization of the Human Nucleus Pulposus Cell Phenotype and Evaluation of Novel Marker Gene Expression to Define Adult Stem Cell Differentiation. Arthritis Rheum. 62, 3695–3705. doi:10.1002/art.27710
Mio, F., Chiba, K., Hirose, Y., Kawaguchi, Y., Mikami, Y., Oya, T., et al. (2007). A Functional Polymorphism in COL11A1, Which Encodes the α1 Chain of Type XI Collagen, Is Associated with Susceptibility to Lumbar Disc Herniation. Am. J. Hum. Genet. 81, 1271–1277. doi:10.1086/522377
Mirza, S. K., and Deyo, R. A. (2007). Systematic Review of Randomized Trials Comparing Lumbar Fusion Surgery to Nonoperative Care for Treatment of Chronic Back Pain. Spine 32, 816–823. doi:10.1097/01.brs.0000259225.37454.38
Moon, H. J., Yurube, T., Lozito, T. P., Pohl, P., Hartman, R. A., Sowa, G. A., et al. (2014). Effects of Secreted Factors in Culture Medium of Annulus Fibrosus Cells on Microvascular Endothelial Cells: Elucidating the Possible Pathomechanisms of Matrix Degradation and Nerve In-Growth in Disc Degeneration. Osteoarthritis and cartilage 22, 344–354. doi:10.1016/j.joca.2013.12.008
Moriguchi, Y., Borde, B., Berlin, C., Wipplinger, C., Sloan, S. R., Kirnaz, S., et al. (2018). In Vivo annular Repair Using High-Density Collagen Gel Seeded with Annulus Fibrosus Cells. Acta Biomater. 79, 230–238. doi:10.1016/j.actbio.2018.07.008
Moskowitz, R. W., Ziv, I., Denko, C. W., Boja, B., Jones, P. K., and Adler, J. H. (1990). Spondylosis in Sand Rats: A Model of Intervertebral Disc Degeneration and Hyperostosis. J. Orthop. Res. 8, 401–411. doi:10.1002/jor.1100080312
Mukasa, D., and Sung, J. (2020). A Prediction Model of Low Back Pain Risk: a Population Based Cohort Study in Korea. Korean J. Pain 33, 153–165. doi:10.3344/kjp.2020.33.2.153
Mulleman, D., Mammou, S., Griffoul, I., Watier, H., and Goupille, P. (2006). Pathophysiology of Disk-Related Sciatica. I.-Evidence Supporting a Chemical Component. Jt. Bone Spine 73, 151–158. doi:10.1016/j.jbspin.2005.03.003
Mundy, C., Yasuda, T., Kinumatsu, T., Yamaguchi, Y., Iwamoto, M., Enomoto-Iwamoto, M., et al. (2011). Synovial Joint Formation Requires Local Ext1 Expression and Heparan Sulfate Production in Developing Mouse Embryo Limbs and Spine. Developmental Biol. 351, 70–81. doi:10.1016/j.ydbio.2010.12.022
Muramatsu, D., Yamaguchi, H., Minamisawa, Y., and Nii, A. (2020). Selective Chemonucleolysis with Condoliase in Cynomolgus Monkeys. Toxicol. Pathol. 48, 656–668. doi:10.1177/0192623320928006
Naba, A., Clauser, K. R., Ding, H., Whittaker, C. A., Carr, S. A., and Hynes, R. O. (2016). The Extracellular Matrix: Tools and Insights for the “Omics” Era. Matrix Biol. 49, 10–24. doi:10.1016/j.matbio.2015.06.003
Nagae, M., Ikeda, T., Mikami, Y., Hase, H., Ozawa, H., Matsuda, K.-I., et al. (2007). Intervertebral Disc Regeneration Using Platelet-Rich Plasma and Biodegradable Gelatin Hydrogel Microspheres. Tissue Eng. 13, 147–158. doi:10.1089/ten.2006.0042
Nakashima, S., Matsuyama, Y., Takahashi, K., Satoh, T., Koie, H., Kanayama, K., et al. (2009). Regeneration of Intervertebral Disc by the Intradiscal Application of Cross-Linked Hyaluronate Hydrogel and Cross-Linked Chondroitin Sulfate Hydrogel in a Rabbit Model of Intervertebral Disc Injury. Biomed. Mater. Eng. 19, 421–429. doi:10.3233/bme-2009-0608
Naqvi, S. M., and Buckley, C. T. (2015). Differential Response of Encapsulated Nucleus Pulposus and Bone Marrow Stem Cells in Isolation and Coculture in Alginate and Chitosan Hydrogels. Tissue Eng. A 21, 288–299. doi:10.1089/ten.tea.2013.0719
Nasto, L. A., Seo, H. Y., Robinson, A. R., Tilstra, J. S., Clauson, C. L., Sowa, G. A., et al. (2012). ISSLS Prize Winner: Inhibition Of NF-κb Activity Ameliorates Age-Associated Disc Degeneration In A Mouse Model Of Accelerated Aging. Spine 37, 1819–1825. doi:10.1097/BRS.0b013e31824ee8f7
Navaro, Y., Bleich-Kimelman, N., Hazanov, L., Mironi-Harpaz, I., Shachaf, Y., Garty, S., et al. (2015). Matrix Stiffness Determines the Fate of Nucleus Pulposus-Derived Stem Cells. Biomaterials 49, 68–76. doi:10.1016/j.biomaterials.2015.01.021
Neubüser, A., Koseki, H., and Balling, R. (1995). Characterization and Developmental Expression of Pax9, a Paired-Box-Containing Gene Related to Pax1. Developmental Biol. 170, 701–716.
Noriega, D. C., Ardura, F., Hernández-Ramajo, R., Martín-Ferrero, M. Á., Sánchez-Lite, I., Toribio, B., et al. (2017). Intervertebral Disc Repair by Allogeneic Mesenchymal Bone Marrow Cells. Transplantation 101, 1945–1951. doi:10.1097/tp.0000000000001484
Nuckley, D. J., Kramer, P. A., Del Rosario, A., Fabro, N., Baran, S., and Ching, R. P. (2008). Intervertebral Disc Degeneration in a Naturally Occurring Primate Model: Radiographic and Biomechanical Evidence. J. Orthop. Res. 26, 1283–1288. doi:10.1002/jor.20526
Nunley, P. D., Coric, D., Frank, K. A., and Stone, M. B. (2018). Cervical Disc Arthroplasty: Current Evidence and Real-World Application. Neurosurgery 83, 1087–1106. doi:10.1093/neuros/nyx579
Oegema, T. R., Johnson, S. L., Aguiar, D. J., and Ogilvie, J. W. (2000). Fibronectin and its Fragments Increase with Degeneration in the Human Intervertebral Disc. Spine 25, 2742–2747. doi:10.1097/00007632-200011010-00005
Ohnishi, T., Yamada, K., Iwasaki, K., Tsujimoto, T., Higashi, H., Kimura, T., et al. (2019). Caspase-3 Knockout Inhibits Intervertebral Disc Degeneration Related To Injury But Accelerates Degeneration Related To Aging. Sci. Rep. 9, 19324. doi:10.1038/s41598-019-55709-3
Önnerfjord, P., Khabut, A., Reinholt, F. P., Svensson, O., and Heinegård, D. (2012). Quantitative Proteomic Analysis of Eight Cartilaginous Tissues Reveals Characteristic Differences as Well as Similarities between Subgroups. J. Biol. Chem. 287, 18913–18924. doi:10.1074/jbc.M111.298968
Orozco, L., Soler, R., Morera, C., Alberca, M., Sánchez, A., and García-Sancho, J. (2011). Intervertebral Disc Repair by Autologous Mesenchymal Bone Marrow Cells: A Pilot Study. Transplantation 92, 822–828. doi:10.1097/tp.0b013e3182298a15
Panebianco, C. J., Dave, A., Charytonowicz, D., Sebra, R., and Iatridis, J. C. (2021). Single-cell RNA-Sequencing Atlas of Bovine Caudal Intervertebral Discs: Discovery of Heterogeneous Cell Populations with Distinct Roles in Homeostasis. FASEB J. : official Publ. Fed. Am. Societies Exp. Biol. 35. doi:10.1096/fj.202101149r
Pattappa, G., Li, Z., Peroglio, M., Wismer, N., Alini, M., and Grad, S. (2012). Diversity of Intervertebral Disc Cells: Phenotype and Function. J. Anat. 221, 480–496. doi:10.1111/j.1469-7580.2012.01521.x
Peacock, A. (1951). Observations on the Prenatal Development of the Intervertebral Disc in Man. J. Anat. 85, 260–274.
Peck, S. H., Mckee, K. K., Tobias, J. W., Malhotra, N. R., Harfe, B. D., and Smith, L. J. (2017). Whole Transcriptome Analysis of Notochord-Derived Cells during Embryonic Formation of the Nucleus Pulposus. Sci. Rep. 7, 10504. doi:10.1038/s41598-017-10692-5
Pereira, C. L., Gonçalves, R. M., Peroglio, M., Pattappa, G., D'este, M., Eglin, D., et al. (2014). The Effect of Hyaluronan-Based Delivery of Stromal Cell-Derived Factor-1 on the Recruitment of MSCs in Degenerating Intervertebral Discs. Biomaterials 35, 8144–8153. doi:10.1016/j.biomaterials.2014.06.017
Perera, K., Ivone, R., Natekin, E., Wilga, C. A., Shen, J., and Menon, J. U. (2021). 3D Bioprinted Implants for Cartilage Repair in Intervertebral Discs and Knee Menisci. Front. Bioeng. Biotechnol. 9, 754113. doi:10.3389/fbioe.2021.754113
Peters, H., Wilm, B., Sakai, N., Imai, K., Maas, R., and Balling, R. (1999). Pax1 and Pax9 Synergistically Regulate Vertebral Column Development. Development (Cambridge, England) 126, 5399–5408. doi:10.1242/dev.126.23.5399
Phillips, F. M., Geisler, F. H., Gilder, K. M., Reah, C., Howell, K. M., and McAfee, P. C. (2015). Long-term Outcomes of the US FDA IDE Prospective, Randomized Controlled Clinical Trial Comparing PCM Cervical Disc Arthroplasty with Anterior Cervical Discectomy and Fusion. Spine 40, 674–683. doi:10.1097/brs.0000000000000869
Piazza, M., Peck, S. H., Gullbrand, S. E., Bendigo, J. R., Arginteanu, T., Zhang, Y., et al. (2018). Quantitative MRI Correlates with Histological Grade in a Percutaneous Needle Injury Mouse Model of Disc Degeneration. J. Orthop. Res. 36, 2771–2779. doi:10.1002/jor.24028
Pirvu, T., Blanquer, S. B. G., Benneker, L. M., Grijpma, D. W., Richards, R. G., Alini, M., et al. (2015). A Combined Biomaterial and Cellular Approach for Annulus Fibrosus Rupture Repair. Biomaterials 42, 11–19. doi:10.1016/j.biomaterials.2014.11.049
Pirvu, T. N., Schroeder, J. E., Peroglio, M., Verrier, S., Kaplan, L., Richards, R. G., et al. (2014). Platelet-rich Plasma Induces Annulus Fibrosus Cell Proliferation and Matrix Production. Eur. Spine J. 23, 745–753. doi:10.1007/s00586-014-3198-x
Pittenger, M. F., Mackay, A. M., Beck, S. C., Jaiswal, R. K., Douglas, R., Mosca, J. D., et al. (1999). Multilineage Potential of Adult Human Mesenchymal Stem Cells. Science 284, 143–147. doi:10.1126/science.284.5411.143
Platenberg, R. C., B. Hubbard, G., J. Ehler, W., and J. Hixson, C. (2001). Spontaneous Disc Degeneration in the Baboon Model: Magnetic Resonance Imaging and Histopathologic Correlation. J. Med. Primatol 30, 268–272. doi:10.1034/j.1600-0684.2001.d01-59.x
Pourquié, O., Coltey, M., Teillet, M. A., Ordahl, C., and Le Douarin, N. M. (1993). Control of Dorsoventral Patterning of Somitic Derivatives by Notochord and Floor Plate. Proc. Natl. Acad. Sci. U S A. 90, 5242–5246. doi:10.1073/pnas.90.11.5242
Priyadarshani, P., Li, Y., and Yao, L. (2016). Advances in Biological Therapy for Nucleus Pulposus Regeneration. Osteoarthritis and Cartilage 24, 206–212. doi:10.1016/j.joca.2015.08.014
Qian, J., Ge, J., Yan, Q., Wu, C., Yang, H., and Zou, J. (2019). Selection of the Optimal Puncture Needle for Induction of a Rat Intervertebral Disc Degeneration Model. Pain Physician 22, 353–360.
Radek, M., Pacholczyk-Sienicka, B., Jankowski, S., Albrecht, Ł., Grodzka, M., Depta, A., et al. (2016). Assessing the Correlation between the Degree of Disc Degeneration on the Pfirrmann Scale and the Metabolites Identified in HR-MAS NMR Spectroscopy. Magn. Reson. Imaging 34, 376–380. doi:10.1016/j.mri.2015.12.005
Raj, P. P. (2008). Intervertebral Disc: Anatomy-Physiology-Pathophysiology-Treatment. Pain Pract. 8, 18–44. doi:10.1111/j.1533-2500.2007.00171.x
Rajasekaran, S., Thangavel, C., Djuric, N., Raveendran, M., Soundararajan, D. C. R., Nayagam, S. M., et al. (2021). Profiling Extra Cellular Matrix Associated Proteome of Human Fetal Nucleus Pulposus in Search for Regenerative Targets. Sci. Rep. 2021, 11111–1901310. doi:10.1038/s41598-021-97620-w
Rajasekaran, S., Babu, J. N., Arun, R., Armstrong, B. R. W., Shetty, A. P., and Murugan, S. (2004). ISSLS Prize Winner: A Study of Diffusion in Human Lumbar Discs: A Serial Magnetic Resonance Imaging Study Documenting the Influence of the Endplate on Diffusion in Normal and Degenerate Discs. Spine 29, 2654–2667. doi:10.1097/01.brs.0000148014.15210.64
Rajasekaran, S., Soundararajan, D. C. R., Tangavel, C., K. S., S. V. A., Nayagam, S. M., Matchado, M. S., et al. (2020). Proteomic Signature of Nucleus Pulposus in Fetal Intervertebral Disc. Asian Spine J. 14, 409–420. doi:10.31616/asj.2019.0217
Reid, J. E., Meakin, J. R., Robins, S. P., Skakle, J. M. S., and Hukins, D. W. L. (2002). Sheep Lumbar Intervertebral Discs as Models for Human Discs. Clin. Biomech. 17, 312–314. doi:10.1016/s0268-0033(02)00009-8
Richardson, S. M., Kalamegam, G., Pushparaj, P. N., Matta, C., Memic, A., Khademhosseini, A., et al. (2016). Mesenchymal Stem Cells in Regenerative Medicine: Focus on Articular Cartilage and Intervertebral Disc Regeneration. Methods 99, 69–80. doi:10.1016/j.ymeth.2015.09.015
Richardson, S. M., Ludwinski, F. E., Gnanalingham, K. K., Atkinson, R. A., Freemont, A. J., and Hoyland, J. A. (2017). Notochordal and Nucleus Pulposus Marker Expression Is Maintained by Sub-populations of Adult Human Nucleus Pulposus Cells through Aging and Degeneration. Sci. Rep. 7, 1501. doi:10.1038/s41598-017-01567-w
Riester, S. M., Lin, Y., Wang, W., Cong, L., Mohamed Ali, A.-M., Peck, S. H., et al. (2018). RNA Sequencing Identifies Gene Regulatory Networks Controlling Extracellular Matrix Synthesis in Intervertebral Disk Tissues. J. Orthop. Res. 36, 1356–1369. doi:10.1002/jor.23834
Risbud, M. V., Schaer, T. P., and Shapiro, I. M. (2010). Toward an Understanding of the Role of Notochordal Cells in the Adult Intervertebral Disc: From Discord to accord. Dev. Dyn. 239, 2141–2148. doi:10.1002/dvdy.22350
Risbud, M. V., and Shapiro, I. M. (2011). Notochordal Cells in the Adult Intervertebral Disc: New Perspective on an Old Question. Crit. Rev. Eukar Gene Expr. 21, 29–41. doi:10.1615/critreveukargeneexpr.v21.i1.30
Roberts, S., Evans, E. H., Kletsas, D., Jaffray, D. C., and Eisenstein, S. M. (2006). Senescence in Human Intervertebral Discs. Eur. Spine J. 15, S312–S316. doi:10.1007/s00586-006-0126-8
Roberts, S., Menage, J., and Urban, J. P. G. (1989). Biochemical and Structural Properties of the Cartilage End-Plate and its Relation to the Intervertebral Disc. Spine 14, 166–174. doi:10.1097/00007632-198902000-00005
Rodrigues-Pinto, R., Ward, L., Humphreys, M., Zeef, L. A. H., Berry, A., Hanley, K. P., et al. (2018). Human Notochordal Cell Transcriptome Unveils Potential Regulators of Cell Function in the Developing Intervertebral Disc. Sci. Rep. 8, 12866. doi:10.1038/s41598-018-31172-4
Rodrigues-Pinto, R., Richardson, S. M., and Hoyland, J. A. (2014). An Understanding of Intervertebral Disc Development, Maturation and Cell Phenotype Provides Clues to Direct Cell-Based Tissue Regeneration Therapies for Disc Degeneration. Eur. Spine J. 23, 1803–1814. doi:10.1007/s00586-014-3305-z
Roughley, P. J., Lamplugh, L., Lee, E. R., Matsumoto, K., and Yamaguchi, Y. (2011). The Role of Hyaluronan Produced by Has2 Gene Expression in Development of the Spine. Spine (Phila Pa 1976) 36, E914–E920. doi:10.1097/BRS.0b013e3181f1e84f
Roughley, P. J. (2004). Biology of Intervertebral Disc Aging and Degeneration. Spine 29, 2691–2699. doi:10.1097/01.brs.0000146101.53784.b1
Russo, F., Ambrosio, L., Peroglio, M., Guo, W., Wangler, S., Gewiess, J., et al. (2021). A Hyaluronan and Platelet-Rich Plasma Hydrogel for Mesenchymal Stem Cell Delivery in the Intervertebral Disc: An Organ Culture Study. Int. J. Mol. Sci. 22. doi:10.3390/ijms22062963
Saal, J. A., and Saal, J. S. (2000a). Intradiscal Electrothermal Treatment for Chronic Discogenic Low Back Pain. Spine 25, 2622–2627. doi:10.1097/00007632-200010150-00013
Saal, J. A., and Saal, J. S. (2002b). Intradiscal Electrothermal Treatment for Chronic Discogenic Low Back Pain. Spine 27, 966–973. doi:10.1097/00007632-200205010-00017
Sahlman, J., Inkinen, R., Hirvonen, T., Lammi, M. J., Lammi, P. E., Nieminen, J., et al. (2001). Premature Vertebral Endplate Ossification And Mild Disc Degeneration In Mice After Inactivation Of One Allele Belonging To The Col2a1 Gene For Type II Collagen. Spine 26, 2558–2565. doi:10.1097/00007632-200112010-00008
Sakai, D., and Andersson, G. B. J. (2015). Stem Cell Therapy for Intervertebral Disc Regeneration: Obstacles and Solutions. Nat. Rev. Rheumatol. 11, 243–256. doi:10.1038/nrrheum.2015.13
Sakai, D., and Schol, J. (2017). Cell Therapy for Intervertebral Disc Repair: Clinical Perspective. J. Orthopaedic Translation 9, 8–18. doi:10.1016/j.jot.2017.02.002
Sarath Babu, N., Krishnan, S., Brahmendra Swamy, C. V., Venkata Subbaiah, G. P., Gurava Reddy, A. V., and Idris, M. M. (2016). Quantitative Proteomic Analysis of normal and Degenerated Human Intervertebral Disc. Spine J. 16, 989–1000. doi:10.1016/j.spinee.2016.03.051
Sarver, J. J., and Elliott, D. M. (2004). Altered Disc Mechanics In Mice Genetically Engineered For Reduced Type I Collagen. Spine 29, 1094–1098. doi:10.1097/00007632-200405150-00009
Schmitt, C., Radetzki, F., Stirnweiss, A., Mendel, T., Ludtka, C., Friedmann, A., et al. (2021). Long‐term Pre‐clinical Evaluation of an Injectable Chitosan Nanocellulose Hydrogel with Encapsulated Adipose‐derived Stem Cells in an Ovine Model for IVD Regeneration. J. Tissue Eng. Regenerative Med. doi:10.1002/term.3216
Schmocker, A., Khoushabi, A., Frauchiger, D. A., Gantenbein, B., Schizas, C., Moser, C., et al. (2016). A Photopolymerized Composite Hydrogel and Surgical Implanting Tool for a Nucleus Pulposus Replacement. Biomaterials 88, 110–119. doi:10.1016/j.biomaterials.2016.02.015
Semba, K., Araki, K., Li, Z., Matsumoto, K., Suzuki, M., Nakagata, N., et al. (2006). A Novel Murine Gene, Sickle Tail, Linked To The Danforth’s Short Tail Locus, is Required For Normal Development Of The Intervertebral Disc. Genetics 172, 445–456. doi:10.1534/genetics.105.048934
Shen, Q., Zhang, L., Chai, B., and Ma, X. (2015). Isolation and Characterization of Mesenchymal Stem-like Cells from Human Nucleus Pulposus Tissue. Sci. China Life Sci.Life Sciences 58, 509–511. doi:10.1007/s11427-015-4839-y
Sheng, B., Feng, C., Zhang, D., Spitler, H., and Shi, L. (2017). Associations between Obesity and Spinal Diseases: A Medical Expenditure Panel Study Analysis. Int. J. Environ. Res. Public Health 14. doi:10.3390/ijerph14020183
Shi, K., Huang, Y., Huang, L., Wang, J., Wang, Y., Feng, G., et al. (2020). Research Progress of Hydrogel Used for Regeneration of Nucleus Pulposus in Intervertebral Disc Degeneration. Zhongguo Xiu Fu Chong Jian Wai Ke Za Zhi 34, 275–284. doi:10.7507/1002-1892.201907092
Silberberg, R., and Gerritsen, G. (1976). Aging Changes in Intervertebral Discs and Spondylosis in Chinese Hamsters. Diabetes 25, 477–483. doi:10.2337/diab.25.6.477
Silva, M. J., and Holguin, N. (2020). Aging Aggravates Intervertebral Disc Degeneration by Regulating Transcription Factors toward Chondrogenesis. FASEB j. 34, 1970–1982. doi:10.1096/fj.201902109r
Simmons, J. W., Upman, P. J., and Stavinoha, W. B. (1984). Pharmacologic and Toxicologic Profile of Chymopapain B (Chemolase). Drug Chem. Toxicol. 7, 299–314. doi:10.3109/01480548409035110
Singh, K., Masuda, K., and An, H. S. (2005). Animal Models for Human Disc Degeneration. Spine J. 5, S267–S279. doi:10.1016/j.spinee.2005.02.016
Singh, V., Manchikanti, L., Calodney, A. K., Staats, P. S., Falco, F. J., Caraway, D. L., et al. (2013). Percutaneous Lumbar Laser Disc Decompression: an Update of Current Evidence. Pain Physician 16, Se229–60. doi:10.36076/ppj.2013/16/se229
Sivakamasundari, V., Kraus, P., Sun, W., Hu, X., Lim, S. L., Prabhakar, S., et al. (2017). A Developmental Transcriptomic Analysis of Pax1 and Pax9 in Embryonic Intervertebral Disc Development. Biol. Open 6, 187–199. doi:10.1242/bio.023218
Sivakamasundari, V., and Lufkin, T. (2012). Bridging the Gap: Understanding Embryonic Intervertebral Disc Development. Cell Dev Biol 1.
Sivan, S. S., Hayes, A. J., Wachtel, E., Caterson, B., Merkher, Y., Maroudas, A., et al. (2014a). Biochemical Composition and Turnover of the Extracellular Matrix of the normal and Degenerate Intervertebral Disc. Eur. Spine J. 23, 344–353. doi:10.1007/s00586-013-2767-8
Sivan, S. S., Wachtel, E., and Roughley, P. (2014b). Structure, Function, Aging and Turnover of Aggrecan in the Intervertebral Disc. Biochim. Biophys. Acta (Bba) - Gen. Subjects 1840, 3181–3189. doi:10.1016/j.bbagen.2014.07.013
Smith, L. J., Nerurkar, N. L., Choi, K.-S., Harfe, B. D., and Elliott, D. M. (2011). Degeneration and Regeneration of the Intervertebral Disc: Lessons from Development. Dis. Model. Mech. 4, 31–41. doi:10.1242/dmm.006403
Smits, P., and Lefebvre, V. (2003). Sox5 And Sox6 Are Required For Notochord Extracellular Matrix Sheath Formation, Notochord Cell Survival And Development Of The Nucleus Pulposus Of Intervertebral Discs. Development 130, 1135–1148. doi:10.1242/dev.00331
Solovieva, S., Kouhia, S., Leino-Arjas, P., Ala-Kokko, L., Luoma, K., Raininko, R., et al. (2004). Interleukin 1 Polymorphisms and Intervertebral Disc Degeneration. Epidemiology 15, 626–633. doi:10.1097/01.ede.0000135179.04563.35
Song, Y.-Q., Karasugi, T., Cheung, K. M. C., Chiba, K., Ho, D. W. H., Miyake, A., et al. (2013). Lumbar Disc Degeneration Is Linked to a Carbohydrate Sulfotransferase 3 Variant. J. Clin. Invest. 123, 4909–4917. doi:10.1172/jci69277
Stafford, D. A., Monica, S. D., and Harland, R. M. (2014). Follistatin Interacts with Noggin in the Development of the Axial Skeleton. Mech. Development 131, 78–85. doi:10.1016/j.mod.2013.10.001
Steinberger, J., and Qureshi, S. (2020). Cervical Disc Replacement. Neurosurg. Clin. North America 31, 73–79. doi:10.1016/j.nec.2019.08.009
Stemple, D. L. (2005). Structure and Function of the Notochord: an Essential Organ for Chordate Development. Development 132, 2503–2512. doi:10.1242/dev.01812
Stern, W. E., and Coulson, W. F. (1976). Effects of Collagenase upon the Intervertebral Disc in Monkeys. J. Neurosurg. 44, 32–44. doi:10.3171/jns.1976.44.1.0032
Stokes, I. A. F., and Iatridis, J. C. (2004). Mechanical Conditions that Accelerate Intervertebral Disc Degeneration: Overload versus Immobilization. Spine 29, 2724–2732. doi:10.1097/01.brs.0000146049.52152.da
Stolworthy, D. K., Fullwood, R. A., Merrell, T. M., Bridgewater, L. C., and Bowden, A. E. (2015). Biomechanical Analysis of the Camelid Cervical Intervertebral Disc. J. Orthopaedic Translation 3, 34–43. doi:10.1016/j.jot.2014.12.001
Stoyanov, J., Gantenbein-Ritter, B., Gantenbein-Ritter, B., Bertolo, A., Aebli, N., Baur, M., et al. (2011). Role of Hypoxia and Growth and Differentiation Factor-5 on Differentiation of Human Mesenchymal Stem Cells towards Intervertebral Nucleus Pulposus-like Cells. eCM 21, 533–547. doi:10.22203/ecm.v021a40
Sugimoto, Y., Takimoto, A., Akiyama, H., Kist, R., Scherer, G., Nakamura, T., et al. (2013). Scx+/Sox9+ Progenitors Contribute to the Establishment of the junction between Cartilage and Tendon/ligament. Development 140, 2280–2288. doi:10.1242/dev.096354
Suri, P., Palmer, M. R., Tsepilov, Y. A., Freidin, M. B., Boer, C. G., Yau, M. S., et al. (2018). Genome-wide Meta-Analysis of 158,000 Individuals of European Ancestry Identifies Three Loci Associated with Chronic Back Pain. Plos Genet. 14, e1007601. doi:10.1371/journal.pgen.1007601
Swank, K. R., Furness, J. E., Baker, E. A., Gehrke, C. K., Biebelhausen, S. P., and Baker, K. C. (2020). Metabolomic Profiling in the Characterization of Degenerative Bone and Joint Diseases. Metabolites 10. doi:10.3390/metabo10060223
Sztrolovics, R., Alini, M., Mort, J. S., and Roughley, P. J. (1999). Age-Related Changes in Fibromodulin and Lumican in Human Intervertebral Discs. Spine 24, 1765. doi:10.1097/00007632-199909010-00003
Tam, V., Chen, P., Yee, A., Solis, N., Klein, T., Kudelko, M., et al. (2020). DIPPER, a Spatiotemporal Proteomics Atlas of Human Intervertebral Discs for Exploring Ageing and Degeneration Dynamics. eLife 9, e64940. doi:10.7554/eLife.64940
Tang, R., Jing, L., Willard, V. P., Wu, C. L., Guilak, F., Chen, J., et al. (2018). Differentiation of Human Induced Pluripotent Stem Cells into Nucleus Pulposus-like Cells. Stem Cel Res Ther 9, 61–12. doi:10.1186/s13287-018-0797-1
Tehranzadeh, J., Ton, J. D., and Rosen, C. D. (2005). Advances in Spinal Fusion. Semin. Ultrasound CT MRI 26, 103–113. doi:10.1053/j.sult.2005.02.007
Thomas, L. (1956). Reversible Collapse of Rabbit Ears after Intravenous Papain, and Prevention of Recovery by Cortisone. J. Exp. Med. 104, 245–252. doi:10.1084/jem.104.2.245
Tilkeridis, C., Bei, T., Garantziotis, S., and Stratakis, C. A. (2005). Association of a COL1A1 Polymorphism with Lumbar Disc Disease in Young Military Recruits. J. Med. Genet. 42, e44. doi:10.1136/jmg.2005.033225
Tomaszewski, K. A., Saganiak, K., Gładysz, T., and Walocha, J. A. (2015). The Biology behind the Human Intervertebral Disc and its Endplates. Folia Morphol. 74, 157–168. doi:10.5603/fm.2015.0026
Torre, O. M., Mroz, V., Bartelstein, M. K., Huang, A. H., and Iatridis, J. C. (2019). Annulus Fibrosus Cell Phenotypes in Homeostasis and Injury: Implications for Regenerative Strategies. Ann. N.Y. Acad. Sci. 1442, 61–78. doi:10.1111/nyas.13964
Troussier, B., Lebas, J. F., Chirossel, J. P., Peocʼh, M., Grand, S., Leviel, J. L., et al. (1995). Percutaneous Intradiscal Radio-Frequency Thermocoagulation. Spine 20, 1713–1718. doi:10.1097/00007632-199508000-00013
Tsaryk, R., Gloria, A., Russo, T., Anspach, L., De Santis, R., Ghanaati, S., et al. (2015). Collagen-low Molecular Weight Hyaluronic Acid Semi-interpenetrating Network Loaded with Gelatin Microspheres for Cell and Growth Factor Delivery for Nucleus Pulposus Regeneration. Acta Biomater. 20, 10–21. doi:10.1016/j.actbio.2015.03.041
Tsingas, M., Ottone, O. K., Haseeb, A., Barve, R. A., Shapiro, I. M., Lefebvre, V., et al. (2020). Sox9 Deletion Causes Severe Intervertebral Disc Degeneration Characterized by Apoptosis, Matrix Remodeling, and Compartment-specific Transcriptomic Changes. Matrix Biol. 94, 110–133. doi:10.1016/j.matbio.2020.09.003
Turel, M. K., Kerolus, M. G., Adogwa, O., and Traynelis, V. C. (2017). Cervical Arthroplasty: What Does the Labeling Say? Neurosurg. Focus 42, E2. doi:10.3171/2016.11.FOCUS16414
Turner, J. A., Ersek, M., Herron, L., Haselkorn, J., Kent, D., Ciol, M. A., et al. (1992). Patient Outcomes after Lumbar Spinal Fusions. Jama 268, 907–911. doi:10.1001/jama.268.7.907
Ukeba, D., Yamada, K., Tsujimoto, T., Ura, K., Nonoyama, T., Iwasaki, N., et al. (2021). Bone Marrow Aspirate Concentrate Combined with In Situ Forming Bioresorbable Gel Enhances Intervertebral Disc Regeneration in Rabbits. JBJS 103, e31. doi:10.2106/jbjs.20.00606
Urban, J. P. G., and Fairbank, J. C. T. (2020). Current Perspectives on the Role of Biomechanical Loading and Genetics in Development of Disc Degeneration and Low Back Pain; a Narrative Review. J. Biomech. 102, 109573. doi:10.1016/j.jbiomech.2019.109573
Urban, J. P. G., Holm, S., Maroudas, A., and Nachemson, A. (1982). Nutrition of the Intervertebral Disc. Clin. orthopaedics Relat. Res. 170, 296–302. doi:10.1097/00003086-198210000-00039
Urban, J. P. G., Holm, S., Maroudas, A., and Nachemson, A. (1977). Nutrition of the Intervertebral Disk. Clin. orthopaedics Relat. Res. 129, 101–114. doi:10.1097/00003086-197711000-00012
Urban, J. P. G., Smith, S., and Fairbank, J. C. T. (2004). Nutrition of the Intervertebral Disc. Spine 29, 2700–2709. doi:10.1097/01.brs.0000146499.97948.52
Urits, I., Capuco, A., Sharma, M., Kaye, A. D., Viswanath, O., Cornett, E. M., et al. (2019). Stem Cell Therapies for Treatment of Discogenic Low Back Pain: a Comprehensive Review. Curr. Pain Headache Rep. 23, 65. doi:10.1007/s11916-019-0804-y
Uysal, O., Arslan, E., Gulseren, G., Kilinc, M. C., Dogan, I., Ozalp, H., et al. (2019). Collagen Peptide Presenting Nanofibrous Scaffold for Intervertebral Disc Regeneration. ACS Appl. Bio Mater. 2, 1686–1695. doi:10.1021/acsabm.9b00062
Van Den Akker, G. G. H., Eijssen, L. M. T., Richardson, S. M., Rhijn, L. W. v., Hoyland, J. A., Welting, T. J. M., et al. (2020). A Membranome-Centered Approach Defines Novel Biomarkers for Cellular Subtypes in the Intervertebral Disc. Cartilage 11, 203–220. doi:10.1177/1947603518764260
Van Den Akker, G. G. H., Koenders, M. I., Van De Loo, F. A. J., Van Lent, P. L. E. M., Blaney Davidson, E., and Van Der Kraan, P. M. (2017). Transcriptional Profiling Distinguishes Inner and Outer Annulus Fibrosus from Nucleus Pulposus in the Bovine Intervertebral Disc. Eur. Spine J. 26, 2053–2062. doi:10.1007/s00586-017-5150-3
Veras, M. A., Lim, Y. J., Kuljanin, M., Lajoie, G. A., Urquhart, B. L., and Séguin, C. A. (2020). Protocol for Parallel Proteomic and Metabolomic Analysis of Mouse Intervertebral Disc Tissues. JOR Spine 3, e1099. doi:10.1002/jsp2.1099
Vujovic, S., Henderson, S., Presneau, N., Odell, E., Jacques, T., Tirabosco, R., et al. (2006). Brachyury, a Crucial Regulator of Notochordal Development, Is a Novel Biomarker for Chordomas. J. Pathol. 209, 157–165. doi:10.1002/path.1969
Wagner, T., Wirth, J., Meyer, J., Zabel, B., Held, M., Zimmer, J., et al. (1994). Autosomal Sex Reversal and Campomelic Dysplasia Are Caused by Mutations in and Around the SRY-Related Gene SOX9. Cell 79, 1111–1120. doi:10.1016/0092-8674(94)90041-8
Wahood, W., Yolcu, Y. U., Kerezoudis, P., Goyal, A., Alvi, M. A., Freedman, B. A., et al. (2020). Artificial Discs in Cervical Disc Replacement: A Meta-Analysis for Comparison of Long-Term Outcomes. World Neurosurg. 134, 598–613. e595. doi:10.1016/j.wneu.2019.10.032
Wallin, J., Wilting, J., Koseki, H., Fritsch, R., Christ, B., and Balling, R. (1994). The Role of Pax-1 in Axial Skeleton Development. Development 120, 1109–1121. doi:10.1242/dev.120.5.1109
Walmsley, R. (1953). The Development and Growth of the Intervertebral Disc. Edinb. Med. J. 60, 341–364.
Wang, B., Wu, Y., Shao, Z., Yang, S., Che, B., Sun, C., et al. (2012). Functionalized Self-Assembling Peptide Nanofiber Hydrogel as a Scaffold for Rabbit Nucleus Pulposus Cells. J. Biomed. Mater. Res. 100A, 646–653. doi:10.1002/jbm.a.33300
Wang, J., Huang, Y., Huang, L., Shi, K., Wang, J., Zhu, C., et al. (2021a). Novel Biomarkers of Intervertebral Disc Cells and Evidence of Stem Cells in the Intervertebral Disc. Osteoarthritis and cartilage 29, 389–401. doi:10.1016/j.joca.2020.12.005
Wang, Y., Zhang, Y., Chen, K., Shao, F., Wu, Y., Guo, C., et al. (2021b). Injectable Nanostructured Colloidal Gels Resembling Native Nucleus Pulposus as Carriers of Mesenchymal Stem Cells for the Repair of Degenerated Intervertebral Discs. Mater. Sci. Eng. 128. doi:10.1016/j.msec.2021.112343
Wei, Q., Zhang, X., Zhou, C., Ren, Q., and Zhang, Y. (2019). Roles of Large Aggregating Proteoglycans in Human Intervertebral Disc Degeneration. Connect. Tissue Res. 60, 209–218. doi:10.1080/03008207.2018.1499731
Wilkinson, D. G., Bhatt, S., and Herrmann, B. G. (1990). Expression Pattern of the Mouse T Gene and its Role in Mesoderm Formation. Nature 343, 657–659. doi:10.1038/343657a0
Williams, F. M. K., Bansal, A. T., Van Meurs, J. B., Bell, J. T., Meulenbelt, I., Suri, P., et al. (2013). Novel Genetic Variants Associated with Lumbar Disc Degeneration in Northern Europeans: a Meta-Analysis of 4600 Subjects. Ann. Rheum. Dis. 72, 1141–1148. doi:10.1136/annrheumdis-2012-201551
Williams, S., Alkhatib, B., and Serra, R. (2019). Development of the Axial Skeleton and Intervertebral Disc. Curr. Top. Dev. Biol. 133, 49–90. doi:10.1016/bs.ctdb.2018.11.018
Wishart, D. S. (2019). Metabolomics for Investigating Physiological and Pathophysiological Processes. Physiol. Rev. 99, 1819–1875. doi:10.1152/physrev.00035.2018
Xu, G., Zhang, C., Zhu, K., Bao, Z., Zhou, P., and Li, X. (2020a). Endoscopic Removal of Nucleus Pulposus of Intervertebral Disc on Lumbar Intervertebral Disc Protrusion and the Influence on Inflammatory Factors and Immune Function. Exp. Ther. Med. 19, 301–307. doi:10.3892/etm.2019.8223
Xu, H., Sun, M., Wang, C., Xia, K., Xiao, S., Wang, Y., et al. (2020b). Growth Differentiation Factor-5-Gelatin Methacryloyl Injectable Microspheres Laden with Adipose-Derived Stem Cells for Repair of Disc Degeneration. Biofabrication 13, 015010. doi:10.1088/1758-5090/abc4d3
Yamada, K. (1962). The Dynamics of Experimental Posture. Experimental Study of Intervertebral Disc Herniation in Bipedal Animals. Tokushima J. Exp. Med. 8, 350–361.
Yan, C., Wang, X., Xiang, C., Wang, Y., Pu, C., Chen, L., et al. (2021). Applications of Functionalized Hydrogels in the Regeneration of the Intervertebral Disc. Biomed. Research International 2021. doi:10.1155/2021/2818624
Yang, C., Chen, Y., Li, Z., Cao, H., Chen, K., Lai, P., et al. (2017). Chondrocyte-Specific Knockout of TSC-1 Leads to Congenital Spinal Deformity in Mice. Biomed. Res. Int. 2017. doi:10.1155/2017/8215805
Yang, J.-J., Li, F., Hung, K.-C., Hsu, S.-H., and Wang, J.-L. (2018). Intervertebral Disc Needle Puncture Injury Can Be Repaired Using a Gelatin-Poly (γ-Glutamic Acid) Hydrogel: an In Vitro Bovine Biomechanical Validation. Eur. Spine J. 27, 2631–2638. doi:10.1007/s00586-018-5727-5
Yang, J., Zhu, Y., Wang, F., Deng, L., Xu, X., and Cui, W. (2020). Microfluidic Liposomes-Anchored Microgels as Extended Delivery Platform for Treatment of Osteoarthritis. Chem. Eng. J. 400. doi:10.1016/j.cej.2020.126004
Yang, X., Jia, H., Xing, W., Li, F., Li, M., Sun, K., et al. (2019). Genetic Variants in COL11A2 of Lumbar Disk Degeneration Among Chinese Han Population. Mol. Genet. Genomic Med. 7, e00524. doi:10.1002/mgg3.524
Ye, D., Liang, W., Dai, L., Zhou, L., Yao, Y., Zhong, X., et al. (2015). Comparative and Quantitative Proteomic Analysis of normal and Degenerated Human Annulus Fibrosus Cells. Clin. Exp. Pharmacol. Physiol. 42, 530–536. doi:10.1111/1440-1681.12386
Yee, A., Lam, M. P. Y., Tam, V., Chan, W. C. W., Chu, I. K., Cheah, K. S. E., et al. (2016). Fibrotic-like Changes in Degenerate Human Intervertebral Discs Revealed by Quantitative Proteomic Analysis. Osteoarthritis and cartilage 24, 503–513. doi:10.1016/j.joca.2015.09.020
Yoon, S.-H., Miyazaki, M., Hong, S.-W., Tow, B., Morishita, Y., Hu, M., et al. (2008). A Porcine Model of Intervertebral Disc Degeneration Induced by Annular Injury Characterized with Magnetic Resonance Imaging and Histopathological Findings. Spi 8, 450–457. doi:10.3171/spi/2008/8/5/450
Yu, J., Peter, C., Roberts, S., and Urban, J. P. G. (2002). Elastic Fibre Organization in the Intervertebral Discs of the Bovine Tail. J. Anat. 201, 465–475. doi:10.1046/j.1469-7580.2002.00111.x
Zeng, C., Yang, Q., Zhu, M., Du, L., Zhang, J., Ma, X., et al. (2014). Silk Fibroin Porous Scaffolds for Nucleus Pulposus Tissue Engineering. Mater. Sci. Eng. C 37, 232–240. doi:10.1016/j.msec.2014.01.012
Zhang, D., Jin, L., Reames, D. L., Shen, F. H., Shimer, A. L., and Li, X. (2013). Intervertebral Disc Degeneration And Ectopic Bone Formation In Apolipoprotein E Knockout Mice. J. Orthop. Res. 31 (2), 210–217. doi:10.1002/JOR.22216
Zhang, H., Wang, L., Park, J. B., Park, P., Yang, V. C., Hollister, S. J., et al. (2009). Intradiscal Injection of Simvastatin Retards Progression of Intervertebral Disc Degeneration Induced by Stab Injury. Arthritis Res. Ther. 11, R172. doi:10.1186/ar2861
Zhang, Y., Xiong, C., Kudelko, M., Li, Y., Wang, C., Wong, Y. L., et al. (2018). Early Onset of Disc Degeneration in SM/J Mice Is Associated with Changes in Ion Transport Systems and Fibrotic Events. Matrix Biol. 70, 123–139. doi:10.1016/j.matbio.2018.03.024
Zhang, Y., Zhang, Z., Chen, P., Ma, C. Y., Li, C., Au, T. Y. K., et al. (2020). Directed Differentiation of Notochord-like and Nucleus Pulposus-like Cells Using Human Pluripotent Stem Cells. Cel Rep. 30, 2791–2806. doi:10.1016/j.celrep.2020.01.100
Zhang, Z., Marson, R. L., Ge, Z., Glotzer, S. C., and Ma, P. X. (2015). Simultaneous Nano- and Microscale Control of Nanofibrous Microspheres Self-Assembled from Star-Shaped Polymers. Adv. Mater. 27, 3947–3952. doi:10.1002/adma.201501329
Zhao, B., Lu, M., Wang, D., Li, H., and He, X. (2016). Genome-Wide Identification of Long Noncoding RNAs in Human Intervertebral Disc Degeneration by RNA Sequencing. Biomed. Res. Int. doi:10.1155/2016/3684875
Zhao, Y. P., Tian, Q. Y., Liu, B., Cuellar, J., Richbourgh, B., Jia, T. H., et al. (2015). Progranulin Knockout Accelerates Intervertebral Disc Degeneration In Aging Mice. Sci. Rep. 16 (5), 9102. doi:10.1038/srep09102
Zhu, C., Li, J., Liu, C., Zhou, P., Yang, H., and Li, B. (2016). Modulation of the Gene Expression of Annulus Fibrosus-Derived Stem Cells Using Poly(ether Carbonate Urethane)urea Scaffolds of Tunable Elasticity. Acta Biomater. 29, 228–238. doi:10.1016/j.actbio.2015.09.039
Keywords: intervertebral disc, animal models, regeneration, development, omics, degeneration, therapies
Citation: Hickman TT, Rathan-Kumar S and Peck SH (2022) Development, Pathogenesis, and Regeneration of the Intervertebral Disc: Current and Future Insights Spanning Traditional to Omics Methods. Front. Cell Dev. Biol. 10:841831. doi: 10.3389/fcell.2022.841831
Received: 22 December 2021; Accepted: 09 February 2022;
Published: 11 March 2022.
Edited by:
Chisa Shukunami, Hiroshima University, JapanReviewed by:
Petra Kraus, Clarkson University, United StatesDanny Chan, The University of Hong Kong, Hong Kong SAR, China
Copyright © 2022 Hickman, Rathan-Kumar and Peck. This is an open-access article distributed under the terms of the Creative Commons Attribution License (CC BY). The use, distribution or reproduction in other forums is permitted, provided the original author(s) and the copyright owner(s) are credited and that the original publication in this journal is cited, in accordance with accepted academic practice. No use, distribution or reproduction is permitted which does not comply with these terms.
*Correspondence: Sun H. Peck, sun.peck@vumc.org
†These authors have contributed equally to this work