Abstract
In the present work, the isochoric heat capacities of pure ethanol and ethanol-based nanofluid (ethanol + diamond) were experimentally studied near the critical point of pure base fluid (ethanol). The measurements were taken using a high temperature, high pressure, nearly constant volume adiabatic calorimeter. The combined expanded (k = 2) uncertainty of the density, temperature, and heat capacity measurements at 95% confidence level is estimated to be 0.2%, 15 mK, and 3%, respectively. For pure base fluid (ethanol), the isochoric heat capacity measurements were taken for two selected isochores of 285.2 and 275.58 kg m−3 (critical) in the one- and two-phase regions near the phase transition and the critical temperatures (514.74 K). The measurements for nanofluid (ethanol + diamond) were taken along the selected near-critical isochore of 284.38 kg m−3 for the concentration of 5 mass% of diamond nanoparticles with size of (3–10) nm in the temperature range from (347 to 537) K. We experimentally found that the phase transition temperature of the ethanol + diamond nanofluid is shifting to high-temperature range (to 526.18 K). Thermal instability of the nanofluid (ethanol + diamond) at high temperatures (around 500 K) and its effect on measured properties was studied.









Similar content being viewed by others
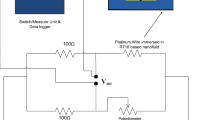
Abbreviations
- T :
-
Temperature/K
- P :
-
Pressure/MPa
- V :
-
Specific volume/m3 kg−1
- \(T_{\text{S}}\) :
-
Phase transition temperature/K
- \(P_{\text{S}}\) :
-
Vapor pressure/MPa
- \(T_{\text{C}}\) :
-
Critical temperature/K
- \(C_{\text{V}}^{{}}\) :
-
Isochoric heat capacity/kJ kg−1 K−1
- \(C_{\text{P}}\) :
-
Isobaric heat capacity/kJ kg−1 K−1
- \(K_{\text{T}}\) :
-
Isothermal compressibility/MPa−1
- m :
-
Mass of a fluid inside the calorimeter/kg
- \(k_{\text{B}}\) :
-
Boltzmann’s constant/J K−1
- \(\Delta Q\) :
-
Electrical energy released by the inner heater/J
- \(U\) :
-
Voltage drops/V
- I :
-
Current passing through the heater/A
- \(C_{ 0}^{{}}\) :
-
Empty calorimeter heat capacity/J K−1
- \(\Delta T\) :
-
Temperature change/K
- \(L_{\text{n}}\) :
-
Average distance between the nanoparticles/nm
- \(n\) :
-
Refraction index
- \(T_{\text{nb}}\) :
-
Normal boiling temperature/K
- \(g_{{{\text{C}}_{\text{V}} }}\) :
-
Finite-size scaling function of heat capacity
- \(x\) :
-
Finite-size scaling variable
- \(V_{{{\text{P}}_{ 0} {\text{T}}_{ 0} }}\) :
-
Volume of the calorimeter at room temperature and 101 kPa m−3
- \(V_{\text{pT}}\) :
-
Volume of the calorimeter at given P and T/m3
- \(\bar{A}_{0}^{ \pm }\) :
-
Asymptotic critical amplitude of heat capacity
- \(\bar{B}_{\text{cr}}\) :
-
Fluctuation-induced “critical background” parameter of heat capacity
- ρ :
-
Density/kg m−3
- \(\rho_{\text{C}}\) :
-
Critical density/kg m−3
- \(\rho_{\text{S}}\) :
-
Saturated density/kg m−3
- \(\rho_{293}\) :
-
Density at 293 K/kg m−3
- τ :
-
Heating time/s
- \(\xi\) :
-
Correlation length/nm
- \(\nu\) :
-
Critical exponent of correlation length
- \(\alpha\) :
-
Critical exponent of heat capacity
- \(\Delta\) :
-
Nonasymptotic critical exponent
- \(\lambda\) :
-
Thermal conductivity/W m−1 K−1
- s :
-
Saturation properties
- c :
-
Critical point
References
McElfresh P, Holcomb D, Ector D. Application of nanofluid technology to improve recovery in oil and gas wells. SPE. 2012;154827:1–6.
McElfresh P, Olguin C, Ector D. The application of nanoparticle dispersions to remove paraffin and polymer filter cake damage. In: SPE 151848, SPE international symposium and exhibition on formation damage control, USA, February 2012; p. 15–7.
McElfresh P, Wood M, Ector D. Stabilizing nanoparticle dispersions in high salinity, high temperature downhole environments. In: SPE 154758, SPE internation oilfiled nanotechnology conference, Noordwijk, The Nitherlands, 12–14 June, 2012; p. 1–6.
Zhang H, Nikolov A, Wasan D. Enhanced oil recovery (EOR) using nanoparticles dispersions: underlying mechanism and Imbibition experiments. Energy Fuels. 2014;28:3002–9.
Wong KV, De Leon O. Applications of nanofluids: current and future. Adv Mech Eng. 2010. https://doi.org/10.1155/2010/519659.
Suleimanov BA, Ismailov FS, Veliyev EF. Nanofluid for enhanced oil recovery. J Pet Sci Eng. 2011;78:431–7.
Oneykonwu MO, Ogolo NA. Investigation the use of nanoparticles in enhancing oil recovery. In: Society for petroleum engineers (SPE) conference paper SPE-140744-MS; 2012. http://dx.doi.org/10.2118/140744-MS.
Onyekonwu M, Ogolo N. Investigating the use of nanoparticles in enhancing oil recovery. In: Nigeria annual international conference and exhibition, Tinapa-Calabar, Nigeria, 31 July–7 August 2010. http://dx.doi.org/10.2118/140744-MS. Accessed 10 June 2017.
Roustaei A, Saffarzadeh S, Mohammadi M. An evaluation of modified silica nanoparticles’ efficiency in enhancing oil recovery of light and intermediate oil reservoirs. Egyp J Pet. 2012;22:427–33.
Karimi A, Fakhroueian Z, Bahramian A, Khiabani NP, Darabad JB, Azin R, Arya S. Wettability alteration in carbonates using zirconium oxide nanofluids: EOR implications. Energy Fuels. 2012;26:1028–36.
Polikhronidi NG, Abdulagatov IM, Magee JW, Stepanov GV. Experimental vapor pressures and derived thermodynamic properties of aqueous solutions of lithium sulfate from 423 to 523 K. Int J Thermophys. 2001;22:189–200.
Polikhronidi NG, Abdulagatov IM, Magee JW, Stepanov GV. Isochoric heat capacity measurements for heavy water near the critical point. Int J Thermophys. 2002;23:745–70.
Polikhronidi NG, Abdulagatov IM, Magee JW, Stepanov GV. Isochoric heat capacity measurements for an equimolar light and heavy water mixture in the near-critical and supercritical regions. Int J Thermophys. 2003;24:405–28.
Polikhronidi NG, Abdulagatov IM, Magee JW, Stepanov GV, Batyrova RG. Isochoric heat capacity measurements for pure methanol in the near-critical and supercritical regions. Int J Thermophys. 2007;28:163–93.
Polikhronidi NG, Batyrova RG, Abdulagatov IM, Magee JW, Stepanov GV. Isochoric heat capacity measurements for a CO2 + n-decane mixture in the near-critical and supercritical regions. J Supercrit Fluids. 2004;33:209–22.
Polikhronidi NG, Batyrova RG, Abdulagatov IM, Stepanov GV. Isochoric heat capacity of CO2 + n-decane mixtures in the critical region. Int J Thermophys. 2006;27:729–59.
Polikhronidi NG, Abdulagatov IM, Stepanov GV, Batyrova RG. Isochoric heat capacity measurements for H2O + CH3OH mixture in the near-critical and supercritical regions. Fluid Phase Equilib. 2007;252:33–46.
Polikhronidi NG, Abdulagatov IM, Batyrova RG, Stepanov GV. Experimental study of the critical behavior of isochoric heat capacity of aqueous ammonia mixture. Int J Thermophys. 2009;30:737–81.
Polikhronidi NG, Batyrova RG, Abdulagatov IM, Magee JW, Wu JT. Saturated and compressed liquid heat capacity at constant volume for 1-hexyl-3-methylimidazolium bis[(trifluoromethyl)sulfonyl]imide). Phys Chem Liq. 2014;52:657–79.
Polikhronidi NG, Batyrova RG, Abdulagatov IM, Magee JW, Wu JT. Thermodynamic properties at saturation derived from experimental two-phase isochoric heat capacity of 1-hexyl-3-methylimidazolium bis[(trifluoromethyl)sulfonyl]imide. Int J Thermophys. 2016;37:103–30.
Polikhronidi NG, Abdulagatov IM, Batyrova RG, Stepanov GV, Wu JT, Ustuzhanin EE. Experimental study of the isochoric heat capacity of diethyl ether (DEE) in the critical and supercritical regions. Int J Thermophys. 2012;33:185–219.
Polikhronidi NG, Abdulagatov IM, Stepanov GV, Batyrova RG. Isochoric heat capacity measurements for pure ethanol in the near-critical and supercritical regions. J Supercrit Fluids. 2007;43:1–24.
Polikhronidi NG, Abdulagatov IM, Batyrova RG. Features of isochoric heat capacity measurements near the phase transition points. Fluid Phase Equilib. 2002;201:269–86.
Abdulagatov IM, Magee JW, Polikhronidi NG, Batyrova RG. In: Letcher T, Wilhelm E, editors. Enthalpy and internal energy: liquids, solutions and vapors. Royal Society of Chemistry; 2017; Chap 15, p. 380–410.
Valyashko VM, Abdulagatov IM. Levelt- Sengers JHM. Vapor-liquid-solid phase transitions in aqueous sodium sulfate and sodium carbonate from heat capacity measurements near the first critical endpoint: part II. The phase boundaries. J Chem Eng Data. 2000;45:1139–49.
Wagner W, Pruß A. The IAPWS formulation 1995 for the thermodynamic properties of ordinary water substance for general and scientific use. J Phys Chem Ref Data. 2002;31:387–535.
Mursalov BA, Abdulagatov IM, Dvoryanchikov VI, Kiselev SB. Isochoric heat capacity of heavy water at sub- and supercritical conditions. Int J Thermophys. 1999;20:1497–528.
Kim SH, Kang JW, Kroenlein K, Magee JM, Diky V, Muzny Ch, Kazakov AF, Chirico RD, Frenkel M. Impact of the uncertainty concept for thermophysical properties. Chem Eng Ed. 2013;47:48–57.
Polikhronidi NG, Abdulagatov IM, Batyrova RG, Stepanov GV. Internal pressure measurements of the binary 0.7393H2O + 0.2607NH3 mixture near the critical and maxcondetherm points. Fluid Phase Equilib. 2010;292:48–57.
Polikhronidi NG, Batyrova RG, Abdulagatov IM, Stepanov GV, Wu JT. PVT and thermal-pressure coefficient measurements of diethyl ether (DEE) in the critical and supercritical ranges. J Chem Thermodyn. 2012;53:67–81.
Polikhronidi NG, Stepanov GV, Abdulagatov IM, Batyrova RG. Thermodynamic study of the n-octane-1-pentanol-sodium dodecyl sulfate solutions in water. Thermochim Acta. 2007;454:99–108.
Polikhronidi NG, Abdulagatov IM, Batyrova RG, Stepanov GV. Experimental study of the thermodynamic properties of diethyl ether (DEE) at the saturation. Int J Thermophys. 2011;32:559–95.
Polikhronidi NG, Abdulagatov IM, Batyrova RG, Stepanov GV. Experimental study of the PVTx properties of aqueous ammonia mixture in the critical and supercritical regions. Int J Refrig. 2009;32:1897–913.
Kamilov IK, Stepanov GV, Abdulagatov IM, Rasulov AR, Milikhina EI. Liquid-liquid-vapor and liquid-vapor phase transitions in aqueous n-hexane mixtures from isochoric heat capacity measurements. J Chem Eng Data. 2001;46:1556–67.
Abdulagatov IM, Polikhronidi NG, Bruno TJ, Batyrova RG, Stepanov GV. Measurements of the isochoric heat capacity, the critical point (T C, ρ C) and vapor-liquid coexistence curve (T S, ρ S) of high-purity toluene near the critical point. Fluid Phase Equilib. 2008;263:71–84.
Schroeder JA. A new fundamental equation for ethanol, Master’s Thesis, University of Idaho; 2011.
Lemmon EW, Huber ML, McLinden MO. NIST standard reference database 23, NIST reference fluid thermodynamic and transport properties, REFPROP, version 9.0, Standard Reference Data Program, National Institute of Standards and Technology: Gaithersburg, MD; 2010.
Nicoll F, Albright PC. Crossover functions by renormalization-group matching: three-loop results. Phys Rev B. 1985;31:4576–89.
Bervillier C. Estimate of a universal critical-amplitude ratio from its ɛ expansion up to ɛ 2. Phys Rev B. 1986;34:8141–3.
Polikhronidi NG, Batyrova RG, Magee JW, Abdulagatov IM. Effect of nanofluids instability on their thermodynamic properties near the critical point. J Chem Thermodyn. 2018; in press (special issue).
Fisher ME. Critical phenomena. In: Hahne FJW, editor. Lectures notes in physics, vol. 186. Berlin: Springer; 1988. p. 1–139.
Fisher ME. The theory of equilibrium critical phenomena. Rep Prog Phys. 1967;30:615–730.
Fisher ME, Zinn S-Y. The shape of the van der waals loop and universal critical amplitude ratios. J Phys A Math Gen. 1998;319:L629–35.
Privman V, Hohenberg PC, Aharony A. In: Domb C, Lebowitz L, editors. Phase transitions and critical phenomena, vol. 14. NY: AP; 1999. p. 1–367.
Fisher ME, Barber MN. Scaling theory for finite-size effects in the critical region. Phys Rev Lett. 1972;28:1516–9.
Garcia-Millan R, Font-Clos F, Corral A. Finite-size scaling of survival probability in branching processes. Centre de Recerca Matemaica, CRM, Preprint # 1200, November 2014.
Elsheikh AH, Sharshir SW, Mostafa ME, Essa FA, Ali MKA. Applications of nanofluids in solar energy: a review of recent advances. Renew Sustain Energy Rev. 2018;82:3483–502.
Leong KY, Ong HC, Amer NH, Norazrina MJ, Risby MS, Ahmad KZ. An overview on current application of nanofluids in solar thermal collector and its challenges. Renew Sustain Energy Rev. 2016;53:1092–105.
Menbari A, Alemrajabi AA, Rezaei A. Heat transfer analysis and the effect of CuO/water nanofluid on direct absorption concentrating solar collector. Appl Thermal Eng. 2016;104:176–83.
Bellos E, Tzivanidis C, Antonopoulos KA, Gkinis G. Thermal enhancements in solar parabolic trough collectors by using nanofluids and converging-diverging absorber tube. Renew Energy. 2016. https://doi.org/10.1016/j.renene.2016.03.062.
Khan MAA, Ibrahim NI, Mahbubul IM, Ali HM, Saidur R, Al-Sulaiman FA. Evaluation of solar collector designs with integrated latent heat thermal energy storage: a review. Sol Energy. 2018;166(166):334–50.
Subramani J, Nagarajan PK, Mahian O, Sathyamurthy R. Efficiency and heat transfer improvements in a parabolic trough solar collector using TiO2 nanofluids under turbulent flow regime. Renew Energy. 2018;119:19–31.
Taylor R, Coulombe S, Otanicar T, Phelan P, Gunawan A, Lv W, Rosengarten G, Prasher R, Tyagi H. Small particles, big impacts: a review of the diverse applications of nanofluids. J Appl Phys. 2013;113:011301.
Abdolbaq MKh, Mamat R, Sidik NAC, Azmi WH, Selvakumar P. Experimental investigation and development of new correlations for heat transfer enhancement and friction factor of BioGlycol/water based TiO2 nanofluids in flat tubes. Int J Heat Mass Trans. 2017;108:1026–35.
Yu W, France DM, Routbort JL, Choi SUS. Review and comparison of nanofluid thermal conductivity and heat transfer enhancements. Heat Transf Eng. 2008;29:432–60.
Sohel Murshed SMS, Nieto de Castro CA. A critical review of traditional and emerging techniques and fluids for electronics cooling. Renew Sustain Energy Rev. 2017;78:821–33.
Das SK, Choi SUS, Patel HE. Heat transfer in nanofluids—a review. Heat Transf Eng. 2006;27:3–19.
Choi SUS. Nanofluids: from vision to reality through research. J Heat Transf. 2009;131:033106.
Saidur R, Leong KY, Mohammad HA. A review on applications and challenges of nanofluids. Renew Sustain Energy Rev. 2011;15:1646–68.
Wang Y, Xu J, Liu Q, Chen Y, Liu H. Performance analysis of a parabolic using Al2O3/synthetic oil nanofluid. App Thermal Eng. 2016;107(469–78):56.
Sohel Murshed SM, Nieto de Castro CA (eds) Nanofluids, synthesis, properties and applications, University of Lisbon, Portugal, NOVA Science Publ. Inc; 2014.
Salgado J, Teijeira T, Parajó JJ, Fernández J, Troncoso J. Isobaric heat capacity of nanostructured liquids with potential use as lubricants. J Chem Thermodyn. 2018;123:107–16.
Zyła G, Vallejo JP, Fal J, Lugo L. Nanodiamonds–ethylene glycol nanofluids: experimental investigation of fundamental physical properties. Int J Heat Mass Trans. 2018;121:1201–13.
Zyła G, Vallejo JP, Lugo L. Isobaric heat capacity and density of ethylene glycol based nanofluids containing, various nitride nanoparticle types: an experimental study. J Mol Liquids. 2018;261:530–9.
Lasfargues M, Geng Q, Cao H, Ding Y. Mechanical dispersion of nanoparticles and its effect on the specific heat capacity of impure binary nitrate salt mixtures. Nanomaterials. 2015;5:1136–46.
Starace AK, Gomez JC, Wang J, Pradhan S, Glatzmaier GC. Nanofluid heat capacities. J Appl Phys. 2011;110:124323. https://doi.org/10.1063/1.3672685.
Hentschke R. On the specific heat capacity enhancement in nanofluids. Nanoscale Res Lett. 2016;11:88–99.
Zhou S-Q, Ni R. Measurement of the specific heat capacity of water-based Al2O3 nanofluid. Appl Phys Lett. 2008;92:093123. https://doi.org/10.1063/1.2890431.
Shin D, Banerjee D. Enhancement of specific heat capacity of high-temperature silica-nanofluids synthesized in alkali chloride salt eutectics for solar thermal-energy storage applications. Int J Heat Mass Trans. 2011;54:1064–70.
Chieruzzi M, Cerritellia GF, Miliozzib A, Kennya JM, Torre L. Heat capacity of nanofluids for solar energy storage produced by dispersing oxide nanoparticles in nitrate salt mixture directly at high temperature. Sol Energy Mater Sol Cells. 2017;167:60–9.
Acknowledgements
This work was supported by Russian Foundation of Basic Research (RFBR) Grants Nos. 16-08-00536 and 18-08-00500.
Author information
Authors and Affiliations
Corresponding author
Rights and permissions
About this article
Cite this article
Polikhronidi, N.G., Batyrova, R.G. & Abdulagatov, I.M. Heat capacity of (ethanol + diamond) nanofluid near the critical point of base fluid (ethanol). J Therm Anal Calorim 135, 1335–1349 (2019). https://doi.org/10.1007/s10973-018-7475-5
Received:
Accepted:
Published:
Issue Date:
DOI: https://doi.org/10.1007/s10973-018-7475-5