Abstract
The branched-chain aminotransferase isozymes BCAT1 and BCAT2, segregated into distinct subcellular compartments and tissues, initiate the catabolism of branched-chain amino acids (BCAAs). However, whether and how BCAT isozymes cooperate with downstream enzymes to control BCAA homeostasis in an intact organism remains largely unknown. Here, we analyse system-wide metabolomic changes in BCAT1- and BCAT2-deficient mouse models. Loss of BCAT2 but not BCAT1 leads to accumulation of BCAAs and branched-chain α-keto acids (BCKAs), causing morbidity and mortality that can be ameliorated by dietary BCAA restriction. Through proximity labelling, isotope tracing and enzymatic assays, we provide evidence for the formation of a mitochondrial BCAA metabolon involving BCAT2 and branched-chain α-keto acid dehydrogenase. Disabling the metabolon contributes to BCAT2 deficiency-induced phenotypes, which can be reversed by BCAT1-mediated BCKA reamination. These findings establish a role for metabolon formation in BCAA metabolism in vivo and suggest a new strategy to modulate this pathway in diseases involving dysfunctional BCAA metabolism.
This is a preview of subscription content, access via your institution
Access options
Access Nature and 54 other Nature Portfolio journals
Get Nature+, our best-value online-access subscription
$29.99 / 30 days
cancel any time
Subscribe to this journal
Receive 12 digital issues and online access to articles
$119.00 per year
only $9.92 per issue
Buy this article
- Purchase on Springer Link
- Instant access to full article PDF
Prices may be subject to local taxes which are calculated during checkout






Similar content being viewed by others
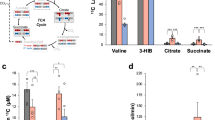
Data availability
All metabolomics datasets are available in Supplementary Tables 1 and 2. The primers and sgRNAs used in this study are listed in Supplementary Table 4. The data supporting the findings of this study are available in the Source data.
References
Hutson, S. M., Sweatt, A. J. & Lanoue, K. F. Branched-chain amino acid metabolism: implications for establishing safe intakes. J. Nutr. 135, 1557s–1564ss (2005).
Neinast, M., Murashige, D. & Arany, Z. Branched chain amino acids. Annu Rev. Physiol. 81, 139–164 (2019).
Lynch, C. J. & Adams, S. H. Branched-chain amino acids in metabolic signalling and insulin resistance. Nat. Rev. Endocrinol. 10, 723–736 (2014).
Sivanand, S. & Vander Heiden, M. G. Emerging roles for branched-chain amino acid metabolism in cancer. Cancer Cell 37, 147–156 (2020).
Brosnan, J. T. & Brosnan, M. E. Branched-chain amino acids: enzyme and substrate regulation. J. Nutr. 136, 207s–211ss (2006).
Suryawan, A. et al. A molecular model of human branched-chain amino acid metabolism. Am. J. Clin. Nutr. 68, 72–81 (1998).
Sweatt, A. J. et al. Branched-chain amino acid catabolism: unique segregation of pathway enzymes in organ systems and peripheral nerves. Am. J. Physiol. Endocrinol. Metab. 286, E64–E76 (2004).
Shimomura, Y., Nanaumi, N., Suzuki, M., Popov, K. M. & Harris, R. A. Purification and partial characterization of branched-chain alpha-ketoacid dehydrogenase kinase from rat liver and rat heart. Arch. Biochem. Biophys. 283, 293–299 (1990).
Damuni, Z., Merryfield, M. L., Humphreys, J. S. & Reed, L. J. Purification and properties of branched-chain alpha-keto acid dehydrogenase phosphatase from bovine kidney. Proc. Natl Acad. Sci. USA 81, 4335–4338 (1984).
Srere, P. A. in Energy Metabolism and the Regulation of Metabolic Processes in Mitochondria (eds Hanson, R. W. and Mehlman, W. A.) 79–91 (Academic Press, 1972).
Srere, P. A. The metabolon. Trends Biochem. Sci. 10, 109–110 (1985).
Islam, M. M. et al. A novel branched-chain amino acid metabolon. Protein-protein interactions in a supramolecular complex. J. Biol. Chem. 282, 11893–11903 (2007).
Islam, M. M. et al. Branched-chain amino acid metabolon: interaction of glutamate dehydrogenase with the mitochondrial branched-chain aminotransferase (BCATm). J. Biol. Chem. 285, 265–276 (2010).
Hutson, S. M., Wallin, R. & Hall, T. R. Identification of mitochondrial branched chain aminotransferase and its isoforms in rat tissues. J. Biol. Chem. 267, 15681–15686 (1992).
Cardoso-Moreira, M. et al. Gene expression across mammalian organ development. Nature 571, 505–509 (2019).
Kim, M. S. et al. A draft map of the human proteome. Nature 509, 575–581 (2014).
Wang, D. et al. A deep proteome and transcriptome abundance atlas of 29 healthy human tissues. Mol. Syst. Biol. 15, e8503 (2019).
Geiger, T. et al. Initial quantitative proteomic map of 28 mouse tissues using the SILAC mouse. Mol. Cell Proteom. 12, 1709–1722 (2013).
Neinast, M. D. et al. Quantitative analysis of the whole-body metabolic fate of branched-chain amino acids. Cell Metab. 29, 417–429.e4 (2019).
Bixel, M., Shimomura, Y., Hutson, S. & Hamprecht, B. Distribution of key enzymes of branched-chain amino acid metabolism in glial and neuronal cells in culture. J. Histochem. Cytochem. 49, 407–418 (2001).
Ding, C. et al. A Cell-type-resolved liver proteome. Mol. Cell Proteom. 15, 3190–3202 (2016).
Walejko, J. M. et al. Branched-chain α-ketoacids are preferentially reaminated and activate protein synthesis in the heart. Nat. Commun. 12, 1680 (2021).
Xia, J., Psychogios, N., Young, N. & Wishart, D. S. MetaboAnalyst: a web server for metabolomic data analysis and interpretation. Nucleic Acids Res. 37, W652–W660 (2009).
Pang, Z. et al. MetaboAnalyst 5.0: narrowing the gap between raw spectra and functional insights. Nucleic Acids Res. 49, W388–w396 (2021).
Chuang, D. T., Shih, V. E. & Max Wynn, R. in The Online Metabolic and Molecular Bases of Inherited Disease (eds Valle, D. L. et al.) (McGraw-Hill Education, 2019).
Blackburn, P. R. et al. Maple syrup urine disease: mechanisms and management. Appl. Clin. Genet. 10, 57–66 (2017).
Snyderman, S. E., Norton, P. M., Roitman, E. & Holt, L. E. Jr. Maple syrup urine disease, with particular reference to dietotherapy. Pediatrics 34, 454–472 (1964).
Homanics, G. E., Skvorak, K., Ferguson, C., Watkins, S. & Paul, H. S. Production and characterization of murine models of classic and intermediate maple syrup urine disease. BMC Med. Genet. 7, 33 (2006).
Zinnanti, W. J. et al. Dual mechanism of brain injury and novel treatment strategy in maple syrup urine disease. Brain 132, 903–918 (2009).
She, P. et al. Disruption of BCATm in mice leads to increased energy expenditure associated with the activation of a futile protein turnover cycle. Cell Metab. 6, 181–194 (2007).
Ananieva, E. A. et al. Mice deficient in the mitochondrial branched-chain aminotransferase (BCATm) respond with delayed tumour growth to a challenge with EL-4 lymphoma. Br. J. Cancer 119, 1009–1017 (2018).
Harper, A. E. & Peters, J. C. Protein intake, brain amino acid and serotonin concentrations and protein self-selection. J. Nutr. 119, 677–689 (1989).
Wu, J. Y. et al. ENU mutagenesis identifies mice with mitochondrial branched-chain aminotransferase deficiency resembling human maple syrup urine disease. J. Clin. Invest. 113, 434–440 (2004).
Paxton, R. & Harris, R. A. Isolation of rabbit liver branched chain alpha-ketoacid dehydrogenase and regulation by phosphorylation. J. Biol. Chem. 257, 14433–14439 (1982).
Branon, T. C. et al. Efficient proximity labeling in living cells and organisms with TurboID. Nat. Biotechnol. 36, 880–887 (2018).
Islam, M. M. et al. A novel branched-chain amino acid metabolon: protein-protein interactions in a supramolecular complex. J. Biol. Chem. 282, 11893–11903 (2007).
Sweetlove, L. J. & Fernie, A. R. The role of dynamic enzyme assemblies and substrate channelling in metabolic regulation. Nat. Commun. 9, 2136 (2018).
Jouvet, P. et al. Branched chain amino acids induce apoptosis in neural cells without mitochondrial membrane depolarization or cytochrome c release: implications for neurological impairment associated with maple syrup urine disease. Mol. Biol. Cell 11, 1919–1932 (2000).
Sgaravatti, A. M. et al. Inhibition of brain energy metabolism by the alpha-keto acids accumulating in maple syrup urine disease. Biochim. Biophys. Acta 1639, 232–238 (2003).
Bridi, R. et al. Alpha-keto acids accumulating in maple syrup urine disease stimulate lipid peroxidation and reduce antioxidant defences in cerebral cortex from young rats. Metab. Brain Dis. 20, 155–167 (2005).
Goodwin, G. W., Zhang, B., Paxton, R. & Harris, R. A. Determination of activity and activity state of branched-chain alpha-keto acid dehydrogenase in rat tissues. Methods Enzymol. 166, 189–201 (1988).
Tso, S. C. et al. Benzothiophene carboxylate derivatives as novel allosteric inhibitors of branched-chain α-ketoacid dehydrogenase kinase. J. Biol. Chem. 289, 20583–20593 (2014).
Hattori, A. et al. Cancer progression by reprogrammed BCAA metabolism in myeloid leukaemia. Nature 545, 500–504 (2017).
Gu, Z. et al. Loss of EZH2 reprograms bcaa metabolism to drive leukemic transformation. Cancer Discov. 9, 1228–1247 (2019).
Schwenk, F., Baron, U. & Rajewsky, K. A cre-transgenic mouse strain for the ubiquitous deletion of loxP-flanked gene segments including deletion in germ cells. Nucleic Acids Res. 23, 5080–5081 (1995).
Schmidt, E. K., Clavarino, G., Ceppi, M. & Pierre, P. SUnSET, a nonradioactive method to monitor protein synthesis. Nat. Methods 6, 275–277 (2009).
Ballard, F. J. & Tomas, F. M. 3-Methylhistidine as a measure of skeletal muscle protein breakdown in human subjects: the case for its continued use. Clin. Sci. (Lond.) 65, 209–215 (1983).
Hunter, R. L. & Markert, C. L. Histochemical demonstration of enzymes separated by zone electrophoresis in starch gels. Science 125, 1294–1295 (1957).
Fottrell, P. F. Functions and applications of isoenzymes. Sci. Prog. 55, 543–559 (1967).
Robinson, J. B. Jr. & Srere, P. A. Organization of Krebs tricarboxylic acid cycle enzymes in mitochondria. J. Biol. Chem. 260, 10800–10805 (1985).
An, S., Kumar, R., Sheets, E. D. & Benkovic, S. J. Reversible compartmentalization of de novo purine biosynthetic complexes in living cells. Science 320, 103–106 (2008).
French, J. B. et al. Spatial colocalization and functional link of purinosomes with mitochondria. Science 351, 733–737 (2016).
Baresova, V. et al. Mutations of ATIC and ADSL affect purinosome assembly in cultured skin fibroblasts from patients with AICA-ribosiduria and ADSL deficiency. Hum. Mol. Genet 21, 1534–1543 (2012).
Wang, X. L., Li, C. J., Xing, Y., Yang, Y. H. & Jia, J. P. Hypervalinemia and hyperleucine-isoleucinemia caused by mutations in the branched-chain-amino-acid aminotransferase gene. J. Inherit. Metab. Dis. 38, 855–861 (2015).
Knerr, I. et al. Expanding the genetic and phenotypic spectrum of branched-chain amino acid transferase 2 deficiency. J. Inherit. Metab. Dis. 42, 809–817 (2019).
Strauss, K. A. et al. Elective liver transplantation for the treatment of classical maple syrup urine disease. Am. J. Transpl. 6, 557–564 (2006).
McKiernan, P. J. et al. Liver transplant for inherited metabolic disease among siblings. Clin. Transpl. 34, e14090 (2020).
Strauss, K. A. et al. Branched-chain α-ketoacid dehydrogenase deficiency (maple syrup urine disease): treatment, biomarkers, and outcomes. Mol. Genet Metab. 129, 193–206 (2020).
Greig, J. A. et al. Muscle-directed AAV gene therapy rescues the maple syrup urine disease phenotype in a mouse model. Mol. Genet Metab. 134, 139–146 (2021).
Miura, H., Quadros, R. M. & Gurumurthy, C. B. Easi-CRISPR for creating knock-in and conditional knockout mouse models using long ssDNA donors. Nat. Protoc. 13, 195–215 (2018).
Gu, Z. et al. Silencing of LINE-1 retrotransposons is a selective dependency of myeloid leukemia. Nat. Genet. 53, 672–682 (2021).
Ravi, V., Jain, A., Mishra, S. & Sundaresan, N. R. Measuring protein synthesis in cultured cells and mouse tissues using the non-radioactive SUnSET assay. Curr. Protoc. Mol. Biol. 133, e127 (2020).
Nofal, M. et al. GCN2 adapts protein synthesis to scavenging-dependent growth. Cell Syst. 13, 158–172.e9 (2022).
Sullivan, L. B. et al. Supporting aspartate biosynthesis is an essential function of respiration in proliferating cells. Cell 162, 552–563 (2015).
Acknowledgements
We thank G. Hoxhaj, S. McBrayer and D. Bezwada for discussions, L. Zacharias for assistance with metabolomics and other Xu laboratory members for technical support. We thank the UTSW Metabolic Phenotyping Core for their analysis of AST, ALT and insulin levels, A. Lemoff at the UTSW Proteomics Core, and the UTSW Pre-Clinical MRI Research Core. Figure 2a was created with BioRender.com. J.X. is a Scholar of The Leukaemia & Lymphoma Society (LLS) and an American Society of Haematology (ASH) Scholar. This work was supported by NIH grants (R01CA230631, R01CA259581, R01DK111430 and R21AI158240 to J.X. and R35CA22044901 to R.J.D.), CPRIT grants (RP180504, RP190417, RP220337 and RP220375 to J.X. and RP180778 to R.J.D.), the DoD grant PR191670 (to D.T.C.) and by the Welch Foundation grant I-1942 (to J.X.).
Author information
Authors and Affiliations
Contributions
M.P. and J.X. conceptualized the study. M.P., Z.G., G.Z., R.M.W., P.K., H.C., F.C., H.V., X.G., Y.Z., M.C., M.N. and J.X. were responsible for the methodology. M.P., Z.G., G.Z., R.M.W., P.K., H.C., F.C. and H.V. carried out the investigation. The original draft was written by M.P. and J.X. Review of the manuscript and editing was done by M.P. and J.X. D.T.C., R.J.D. and J.X. were responsible for funding acquisition. Supervision was carried out by D.T.C., R.J.D. and J.X.
Corresponding author
Ethics declarations
Competing interests
M.P. and J.X. have a pending patent application for the use of BCAT isozymes to treat metabolic disorders. M.P., J.X., and The University of Texas Southwestern Medical Center have a financial interest in the pending patent application.
Peer review
Peer review information
Nature Metabolism thanks Cholsoon Jang, Christopher Newgard and the other, anonymous, reviewer(s) for their contribution to the peer review of this work. Primary Handling Editor: Alfredo Giménez-Cassina, in collaboration with the Nature Metabolism team.
Additional information
Publisher’s note Springer Nature remains neutral with regard to jurisdictional claims in published maps and institutional affiliations.
Extended data
Extended Data Fig. 1 Distinct Expression Patterns of BCAT Isozymes in Human and Mouse Tissues.
(a) Expression of BCAT1 mRNA in seven human organs across developmental time. (b) Expression of BCAT2 mRNA in seven human organs across developmental time. (c) Expression correlation of genes encoding enzymes involved in the BCAA metabolic pathway in human tissues across developmental times. The colour and size of each circle indicate the Pearson’s correlation coefficient scores for positive (red) and negative (blue) correlations. P values by a two-sided t-distribution with n-2 degrees of freedom. (d) mRNA and protein expression for BCAT1 and BCAT2 across human tissues. Heatmap shows the normalized expression values for mRNA (pink) and protein (brown) in the GTEx database. (e) Expression of Bcat2, Bckdha, Bckdhb, Bckdk and Ppm1k mRNA in fractionated liver cell types including hepatocytes (HC), hepatic stellate cells (HSC), Kupffer cells (KC), and liver sinusoidal endothelial cells (LSEC). mRNA expression values by RNA-seq (FKPM) are shown. (f) Expression of BCAT2, BCKDHA, BCKDHB, BCKDK and PPM1K protein in fractionated liver cell types. Protein expression values by quantitative proteomics (fraction of total) are shown.
Extended Data Fig. 2 Phenotypic Analysis of BCAT1 and BCAT2 Knockout Mice.
(a) Schematic of the genotyping PCR for Bcat1−/− and Bcat2−/− constitutive KO mice. The locations and sizes of the genotyping primers and PCR products are shown. (b) Representative genotyping PCR and Sanger sequencing results for Bcat1−/− and Bcat2−/− mice. (c) Validation of Bcat1−/− KO by Western blot analysis of mouse brain and heart. HEK293T cells with BCAT1 and BCAT2 double knockout (DKO) and DKO cells with BCAT1 or BCAT2 overexpression (BCAT1OE and BCAT2OE) were analysed as controls. (d) Representative images for male and female mice of the indicated genotypes. (e) Representative H&E staining of epididymal adipose tissue in WT and Bcat2−/− mice. Scale bar, 50 μm. (f) The organ weight relative to body weight is shown for heart, kidney and spleen of WT, Bcat1−/− and Bcat2−/− mice with the number (N) of samples shown. Results are mean ± SD and analysed by one-way ANOVA. *P < 0.05, n.s. not significant. (g) Hindlimb skeletal muscle and epididymal adipose weight of the indicated genotypes. Results are mean ± SD (N = 4 WT and 3 Bcat2−/− mice for muscle, and 4 WT and 4 Bcat2−/− mice for fat) and analysed by two-sided unpaired t-test. (h) Plasma AST and ALT levels in mice of the indicated genotypes. Results are mean ± SD (N = 5 WT and 4 Bcat2−/− mice) and analysed by two-sided unpaired t-test. (i-k) Representative H&E staining of heart (i), kidney (j), and liver (k) from WT or Bcat2−/− mice. Scale bar, 50 μm.
Extended Data Fig. 3 Metabolomic Alterations Caused by BCAT1 or BCAT2 Deficiency.
(a) Principle component analysis of metabolic profiles of liver, pancreas, plasma and muscle in WT, Bcat1−/− and Bcat2−/− mice. (b) Enriched pathways associated with increased or decreased metabolites across major organs in Bcat1−/− mice. Colour scale indicates negative log10 transformed P values of the pathways associated with increased (red) or decreased (blue) metabolites determined by MetaboAnalyst 5.0 pathway analysis using hypergeometric test. (c) Enriched pathways associated with increased or decreased metabolites across major organs in Bcat2−/− mice. Colour scale indicates negative log10 transformed P values of the pathways associated with increased (red) or decreased (blue) metabolites determined by MetaboAnalyst 5.0 pathway analysis using hypergeometric test.
Extended Data Fig. 4 Differentially Enriched Metabolites Caused by BCAT1 or BCAT2 Deficiency.
Hierarchical clustering heatmaps are shown for the top 50 differentially enriched metabolites in each indicated tissue between WT, Bcat1−/− and Bcat2−/− mice from one-way ANOVA analysis. The red and blue colours indicate higher and lower metabolite abundance by MetaboAnalyst 5.0, respectively.
Extended Data Fig. 5 Neuropathology of Bcat2−/− mice.
(a) Transverse relaxation time (T2) maps of WT (left) and Bcat2−/− (right) mouse brains. Colour bar indicates T2 values. (b) Relative abundance of L-DOPA, threonine, tryptophan, and tyrosine in brain tissue of Bcat2−/− mice. Results are mean ± SD (N = 4 WT and 4 Bcat2−/−) and analysed by two-sided unpaired t-test. (c, d) Representative H&E staining of the striatum (c) and brainstem (d) in WT and Bcat2−/− mice. Scale bar, 50 μm.
Extended Data Fig. 6 Generation of BCAT1 or BCAT2 Reconstituted Cell Models for Proximity Labelling.
(a) Western blot analysis of enzymes in the BCAA metabolic pathway in major tissues of WT and Bcat2−/− mice. (b) Western blot analysis and quantification of BCKDHA and P-BCKDHA in WT and Bcat2−/− liver samples. Results shown as mean ± SD (N = 12 WT and 11 Bcat2−/− mice) and analysed by two-sided unpaired t-test. (c) Generation of BCAT1 or BCAT2 reconstituted HEK293T cells. Western blot analysis is shown for the mitochondrial location of mTD, BCAT1-mTD and BCAT2-mTD, and efficient biotinylation of mitochondrial proteins in the presence of doxycycline (Dox) and exogenous biotin. MT-CO2 and p70 S6 kinase (P70-S6K) were analysed as controls for the fractionated mitochondria and cytoplasm, respectively. (d) Validation of efficient biotinylation of mitochondrial proteins by proximity labelling using mTD, BCAT1-mTD or BCAT2-mTD in the presence of Dox and exogenous biotin. (e) Co-immunoprecipitation of BCAT1 and BCKDH in mouse kidney mitochondrial lysates using α-BCKDHA antibody cross-linked Dynabeads Protein A. Input or immunoprecipitated samples were blotted with α-BCKDHA and α-BCAT2 antibodies, respectively. Co-immunoprecipitation was performed in the presence or absence of additives (Val, PLP, α-KG, thiamine diphosphate and Coenzyme A), whereas co-immunoprecipitation using Dynabeads Protein A without cross-linking with α-BCKDHA antibody was performed as a negative control.
Extended Data Fig. 7 Ectopic BCAT1 Expression Ameliorates BCAT2 Deficiency-Induces Metabolic Defects.
(a) Western blot analysis to validate the cytosolic expression of BCAT1 in WT and Bcat1OE kidney lysates. (b) Western blot results are shown for the validation of Bcat1OE and Bcat1OE;Bcat2−/− mice and the expression of enzymes in the BCAA metabolic pathway in major tissues. (c) Fractional enrichment of KIV_M + 5 in the plasma of the indicated genotypes during the 2-hour stable isotope infusion experiments. Results are mean ± SD (N = 4 WT, 4 Bcat2−/−, 3 Bcat1OE and 3 Bcat1OE;Bcat2−/− mice). (d) Relative abundance of Val_M + 0,5 and KIV_M + 0,5 in plasma samples before (0 hour) and after (2 hour) KIV_M + 5 stable isotope infusion with the number (N) of independent samples shown. Results are mean ± SD and analysed by two-way ANOVA. (e) Glutamate/α-KG ratios in major tissues of the indicated genotypes with the number (N) of independent samples shown. Results are mean ± SD and analysed by one-way ANOVA. (f) NAD+/NADH ratio in major organs of the indicated genotypes with the number (N) of samples shown. Results are mean ± SD and analysed by two-way ANOVA. (g) Insulin levels in the serum of the indicated genotypes. Results are mean ± SD (N = 5 WT, 4 Bcat2−/−, 4 Bcat1OE and 4 Bcat1OE;Bcat2−/− mice) and analysed by one-way ANOVA.
Extended Data Fig. 8 BCAT1-Catalysed BCKA Reamination Increases BCAA Transport and Protein Synthesis.
(a) Western blot analysis of LAT1 (encoded by Slc7a5) in major tissues of WT, Bcat1OE and Bcat1OE;Bcat2−/− mice. (b) Quantification of LAT1 expression in mice of the indicated genotypes relative to WT samples with the number (N) of samples shown. Results are mean ± SD and analysed by two-sided unpaired t-test. (c) Representative Western blot analysis of 4EBP1, P-4EBP1, p70S6K, and P-p70S6K in WT or Bcat2−/− liver lysates. Quantification is shown on the bottom. Results are mean ± SD (N = 3 WT and 3 Bcat2−/− mice on BCAA-normal diet, and 3 Bcat2−/− mice on BCAA-choice diet) and analysed by one-way ANOVA. (d) Representative Western blot analysis of 4EBP1, P-4EBP1, p70S6K, and P-p70S6K in liver lysates from mice of the indicated genotypes. Quantification is shown on the bottom. Results are mean ± SD (N = 3 WT, 3 Bcat2−/−, 3 Bcat1OE and 3 Bcat1OE;Bcat2−/− mice) and analysed by one-way ANOVA. (e) Amino acid levels in Bcat2−/−, Bcat1OE, and Bcat1OE;Bcat2−/− mouse tissues. Heatmap shows the log2 fold changes of the indicated metabolites in each mutant sample relative to WT controls (N = 3 Bcat2−/−, 3 Bcat1OE, and 4 Bcat1OE;Bcat2−/− mice). (f) Representative Western blot analysis of puromycin incorporation in major tissues of WT, Bcat1OE and Bcat1OE;Bcat2−/− mice.
Supplementary information
Supplementary Table 1
Metabolomic profiling of BCAT1 and BCAT2 KO mice. The name, mass, HMDB, associated KEGG pathway, fold change relative to WT, raw P value and FDR-corrected P values are shown for each metabolite in the indicated mouse genotype and tissue type.
Supplementary Table 2
Metabolomic profiling of BCAT2 KO with normal or BCAA-choice diet. The name, mass, HMDB, associated KEGG pathway, fold change relative to WT, raw P value and FDR-corrected P values are shown for each metabolite in the indicated mouse with BCAA-normal or BCAA-choice diet.
Supplementary Table 3
Proteins and peptides identified by proximity labelling proteomics. The peptide sequence, modifications and number of PSMs are shown for each identified protein by BCAT2-mTD-mediated proximity labelling.
Supplementary Table 4
List of primers and sgRNAs.
Supplementary Table 5
Statistical analysis of Fig. 6e.
Source data
Source Data Fig. 1
Unprocessed western blots.
Source Data Fig. 1
Statistical source data.
Source Data Fig. 2
Statistical source data.
Source Data Fig. 3
Statistical source data.
Source Data Fig. 4
Unprocessed western blots.
Source Data Fig. 5
Statistical source data.
Source Data Fig. 6
Statistical source data.
Source Data Extended Data Fig. 1
Unprocessed western blots and gels.
Source Data Extended Data Fig. 2
Statistical source data.
Source Data Extended Data Fig. 5
Statistical source data.
Source Data Extended Data Fig. 6
Unprocessed western blots.
Source Data Extended Data Fig. 6
Statistical source data.
Source Data Extended Data Fig. 7
Unprocessed western blots.
Source Data Extended Data Fig. 7
Statistical source data.
Source Data Extended Data Fig. 8
Unprocessed western blots.
Source Data Extended Data Fig. 8
Statistical source data.
Rights and permissions
Springer Nature or its licensor (e.g. a society or other partner) holds exclusive rights to this article under a publishing agreement with the author(s) or other rightsholder(s); author self-archiving of the accepted manuscript version of this article is solely governed by the terms of such publishing agreement and applicable law.
About this article
Cite this article
Patrick, M., Gu, Z., Zhang, G. et al. Metabolon formation regulates branched-chain amino acid oxidation and homeostasis. Nat Metab 4, 1775–1791 (2022). https://doi.org/10.1038/s42255-022-00689-4
Received:
Accepted:
Published:
Issue Date:
DOI: https://doi.org/10.1038/s42255-022-00689-4
This article is cited by
-
A spatiotemporal proteomic map of human adipogenesis
Nature Metabolism (2024)
-
Cellular forgetting, desensitisation, stress and ageing in signalling networks. When do cells refuse to learn more?
Cellular and Molecular Life Sciences (2024)
-
Enhanced BCAT1 activity and BCAA metabolism promotes RhoC activity in cancer progression
Nature Metabolism (2023)
-
BCAT2–BCKDH metabolon maintains BCAA homeostasis
Nature Metabolism (2022)