- 1Instituto de Virología e Innovaciones Tecnológicas, Centro de Investigaciones en Ciencias Veterinarias y Agronómicas, INTA-CONICET, Castelar, Argentina
- 2Departamento de Ciencia y Tecnología, CONICET, Universidad Nacional de Quilmes, Bernal, Argentina
- 3Instituto de Estudios Inmunológicos y Fisiopatológicos (IIFP-CONICET-UNLP), La Plata, Argentina
- 4Poultry Diagnostic and Research Center, College of Veterinary Medicine, University of Georgia, Athens, GA, United States
In chickens, infections due to influenza A virus (IAV) can be mild to severe and lethal. The study of IAV infections in poultry has been mostly limited to strains from the North American and Eurasian lineages, whereas limited information exists on similar studies with strains from the South American lineage (SAm). To better evaluate the risk of introduction of a prototypical SAm IAV strain into poultry, chickens were infected with a wild-type SAm origin strain (WT557/H6N2). The resulting virus progeny was serially passaged in chickens 20 times, and the immunopathological effects of the last passage virus, 20Ch557/H6N2, in chickens were compared to those of the parental strain. A comparison of complete viral genome sequences indicated that the 20Ch557/H6N2 strain contained 13 amino acid differences compared to the wild-type strain. Five of these mutations are in functionally relevant regions of the viral surface glycoproteins hemagglutinin (HA) and neuraminidase (NA). However, despite higher and more prolonged virus shedding in chickens inoculated with the 20Ch557/H6N2 strain compared to those that received the WT557/H6N2 strain, transmission to naïve chickens was not observed for either group. Analyses by flow cytometry of mononuclear cells and lymphocyte subpopulations from the lamina propria and intraepithelial lymphocytic cells (IELs) from the ileum revealed a significant increase in the percentages of CD3+TCRγδ+ IELs in chickens inoculated with the 20Ch557/H6N2 strain compared to those inoculated with the WT557/H6N2 strain.
Introduction
Influenza A virus are enveloped viruses and belong to the family Orthomyxoviridae containing a segmented negative sense single-stranded RNA that encodes for 10 major ORFs and additional 2 – 4 minor ORFs whose expression depends on virus origin and/or strain. Based on the antigenic properties of the viral surface glycoproteins, 18 HA (H1-18) and 11 NA (N1-N11) IAV subtypes have been identified in nature in multiple combinations. Except for the H17N10 and H18N11 IAVs identified in fruit bat species of Guatemala and Peru, (Tong et al., 2012, 2013) respectively, the remaining IAV subtypes are found in wild aquatic birds of the order Anseriformes and Charadriiformes. The spread and diversity of IAVs across the globe depends on the migratory patterns of wild birds that include both regional and intercontinental movement (Olsen et al., 2006). From this primordial reservoir, IAVs with increased host range emerge and establish lineages in a wide range of avian and mammalian species. Most notably, the emergence of IAV in poultry, pigs, horses, and humans has been invariably associated with epidemic and/or pandemic episodes with significant loss of life and/or economic disruptions (Prager et al., 2017). Based on the pathogenesis in chickens and/or the presence of a polybasic amino acid cleavage site in the HA, IAVs that affect poultry are classified into two pathotypes low pathogenicity avian influenza viruses (LPAIVs) and high pathogenicity avian influenza viruses (HPAIVs). Only viruses of the H5 and H7 subtypes appear to be associated with either LPAIV or HPAIV strains, whereas other subtypes are typically characterized as LPAIVs (Abdelwhab et al., 2013). The polybasic cleavage site in HPAIV enables the HA to be processed by endogenous cellular furin-like proteases enabling systemic infection. LPAIVs contain typically a monobasic cleavage site that limits HA processing to extracellular trypsin-like proteases, thus restraining the infection to mostly the intestinal and/or respiratory tract.
In Argentina, active influenza virus surveillance in wild birds was initiated in 2006 (Pereda et al., 2008) as an integral part of worldwide efforts to better understand the ecology of these viruses and to better monitor the potential emergence of strains of concern for poultry, livestock, and humans. These studies led to the realization of IAVs in wild birds in Argentina have unique evolutionary patterns and the presence of a dominant gene segment constellation defined as South American lineage (SAm) (Rimondi et al., 2018). IAVs of the H1, H4, H5, H7, H9, H10, and H13 subtypes were identified in Argentina, but the most common so far is the H6 subtype (∼41%) associated with the N2 subtype. Due to this observation and reports of H6 subtype IAV outbreaks in domestic poultry in Eurasia, North America, and Africa (Hoffmann et al., 2000; Webby et al., 2002; Abolnik et al., 2007; Huang et al., 2010), the prototypic influenza A/rosy-billed pochard/Argentina/CIP051-557/2007 (H6N2) strain, hereafter WT557/H6N2, was selected to establish its replication and pathogenic capacity in chickens. The resulting progeny was adapted through serial passage 20 times in chickens, and the resulting 20Ch557/H6N2 virus was also evaluated for replication and pathogenesis.
Materials and Methods
Viruses and Inoculums
The A/rosy-billed pochard/Argentina/CIP051-557/2007 (H6N2) virus (WT557/H6N2) was isolated from waterfowl as described previously (Rimondi et al., 2011). Stock of WT557/H6N2 virus was prepared by inoculation of embryonated chicken eggs. The chicken-adapted (20Ch557/H6N2) virus was isolated from the 20th chicken lung passage of the WT557/H6N2 virus using the methodology described by Hossain et al. (2008) Stock of 20Ch557/H6N2 virus was prepared by the inoculation of lung homogenates into the allantoic cavity of 10-day-old embryonated chicken eggs.
Chickens and Housing
Specific-pathogen-free (SPF) White Leghorn embryonated eggs (Rosenbusch S.A. CABA, Argentina) were purchased, incubated, and hatched in an automatic incubator (Yonar, CABA, Argentina) under appropriate conditions, and randomly separated into groups of 10 chickens. Groups were housed separately in sterilized isolators for chickens under negative pressure conditions (Allentown CH8ISOL) with food and water ad libitum throughout the experimental period. Animal care and experimental procedures were performed under ABSL3+ conditions with investigators wearing appropriate protective equipment and in accordance with the approved protocols N° 55/2013 of the National Institute of Agricultural Technology Ethics Committee (INTA, Argentina).
Replication and Transmission Studies With H6N2 Viruses
3-weeks old SPF White Leghorn chickens were used throughout the studies. Groups of chickens were inoculated intraocularly, intranasally, intratracheally, and orally with WT557/H6N2 or 20Ch557/H6N2 virus containing 5 × 106 EID50 in 1 ml or 1 ml PBS, respectively. At 1 dpi, four naive chickens were placed together with the infected birds to monitor transmission. Tracheal and cloacal swabs were collected at 1, 3, 5, and 7 dpi in 1 ml freezing medium (50% glycerol in PBS containing 1% antibiotics) and stored at −80°C until use. Virus titers in the swabs were determined by equivalent EID50/ml as previously described (Bertran et al., 2013). The limit of detection was determined to be 102 EID50/ml; therefore, RT-qPCR negative samples were treated as ≤102 EID50/ml. Chickens were observed daily for signs of disease (monitored for appetite, activity, fecal output, and signs of distress including cyanosis of the tongue or legs, ruffled feathers, and respiratory distress), and serum samples were collected at 21 dpi.
To investigate the replication of WT557/H6N2 and 20Ch557/H6N2 viruses in the tissues of chickens (lung and intestine), six birds per group were euthanized using manual cervical dislocation at 3 dpi. This was performed only after chickens were anesthetized with isoflurane. Body and bursa of Fabricius were weighed and recorded in all euthanized animals (six birds/group at 3 dpi).
Epithelial Cells Isolation From the Ileum of Chickens
Single cell suspensions of 10 centimeters of ileum were prepared as described previously (Stringa et al., 2021). Briefly, intestinal sections from the ileum were rinsed, cut longitudinally, and washed with cold PBS containing 1% antibiotics. Following several washes, tissue sections were treated for 30 min at room temperature with PBS and 10 mM EDTA with continuous shaking. This was repeated and supernatants from both incubations were collected after centrifugation at 500 rpm for 3 min. Cells from supernatants were collected by centrifugation at 1,200 rpm for 15 min and resuspended in RPMI 1640 medium. Finally, cells were passed through a 80 μm mesh (Cell Strainer, BD) and IELs were counted using trypan blue exclusion. For the lamina propria compartment, ileum from chickens was cut mechanically and incubated with RPMI 1640 medium with Collagenase II in a concentration of 1 mg/ml (Gibco) for 40 min at 37°C with a shaker. Cells from supernatants were collected by centrifugation at 1,200 rpm for 15 min and resuspended in RPMI 1640 medium. Finally, cells were passed through a 80 μm mesh (Cell Strainer, BD) and lymphocytes were counted using trypan blue exclusion.
Flow Cytometry Analysis
Cells were diluted in staining buffer (PBS 1 ×, 2 % FBS, 10 mM EDTA) and 1 × 106 cells per well were seeded on 96 well-plates (V-shape) and washed 2 times with the same buffer. Staining was performed by resuspending the cellular pellet of each well with 100 μl of staining buffer including different combinations of antibodies, or as single-color staining for compensation. Cells were incubated at 4°C for 20 min and washed 2 times with staining buffer by centrifugation at 1,200 rpm for 3 min.
Monoclonal antibodies (mAbs) (CD3-SPRD, CD4-FITC, CD8α-FITC, CD8α-PE, TCRγδ-PE, BU1-FITC, and KUL01-PE) were purchased from Southern Biotech. (Birmingham, AL), CD25-Alexa 647 was purchased from AbD Serotec (California, United States) and CD56-Alexa 647 was purchased from LSBio (LifeSpan BioSciences, Inc., Seattle, United States). Lymphocytes from lamina propria and epithelial cell compartments from the ileum were stained with three mAb mix: CD3-SPRD, CD4-FITC, CD8α-PE, and CD25-Alexa 647; CD3-SPRD, CD8α-FITC, TCRγδ-PE, and CD25-Alexa 647; and CD3-SPRD, BU1-FITC, KUL01-PE, and CD56-Alexa 647. All antibodies were titrated to determine the optimal staining concentration of each one.
Positive cells were analyzed with a FACS Calibur flow cytometer (BD Biosciences, San Jose, CA) and CellQuest software. Analyses were done on 20,000 events and discrete viable lymphoid cell populations were gated according to the forward/side scatter characteristics. Percentages of different lymphoid cell subpopulations in the ileum were determined by multiparametric analysis.
Serology
Sera were tested by competitive enzyme-linked immunosorbent assay (ELISA) for specific antibodies against Influenza A Virus according to the manufacturer’s recommendations (IDEXX Laboratories Inc., Westbrook, ME) using 10-fold dilutions of each serum sample.
HI Assay
Serum samples were collected to determine the antigenic relatedness between WT557/H6N2 and 20Ch557/H6N2 viruses at the end of the experiment. Sera were treated with receptor destroying enzyme (Accurate Chemical and Scientific Corp., Westbury, NY, United States) to remove sialic acid receptors. The anti-viral antibody titers developed were evaluated using the HI assay system outlined by the WHO Manual on Animal Influenza Diagnosis and Surveillance (Webster et al., 2002). Homologous and heterologous antibody responses were measured by HI assays using the viruses used in the infection experiment.
Amplification and Sequencing of H6N2 Viruses
Total RNA was extracted from the stock virus in an allantoic fluid using the QIAamp Viral RNA Mini Kit (Qiagen Inc., Valencia, CA, United States) according to the manufacturer’s instructions. Reverse transcription and PCR amplification were performed using the universal primers described by Hoffmann et al. (2001). PCR products were purified with a QIAQuick PCR purification kit (Qiagen) and sequencing was performed using the BigDye Terminator v3.1 Cycle Sequencing Kit on an ABI PRISM 3700 DNA Analyzer (Applied Biosystems) following the manufacturer’s instructions.
The consensus amino acid and nucleotide sequences for all eight gene segments of WT557/H6N2 and 20Ch557/H6N2 viruses were generated using BioEdit 7.
Nucleotide Sequence Accession Numbers
The genome sequences obtained in this study for WT557/H6N2 and 20Ch557/H6N2 stock viruses are available from GenBank under accession numbers CY067691 to CY067698 and ON306370 to ON306377.
Statistical Analysis
Data were tested for normal distribution (Shapiro–Wilk test) and homogeneity of variance (Leven’s test) prior to analysis using Statistical Package for the Social Sciences for Windows (SPSS, version 15.0, Chicago, IL, United States.). An ANOVA test was used, and a Tukey’s test was applied when differences were detected. Variables that did not fit the ANOVA’s assumptions were analyzed by Kruskall–Wallis and Dunn’s test. p < 0.05 were considered statistically significant.
Chi-Square tests were used to compare the number of animals that were positive for IAV between different experimental groups.
Results
Serial Passage in Chickens Improves Replication of a Wild Bird Origin H6N2 SAm Influenza A Virus
We followed a strategy that was previously described for adaptation of a domestic duck origin H9N2 virus to Japanese quail and chickens using lung homogenates from the previous passage (Hossain et al., 2008). In this case, we attempted adaptation in chickens of a H6N2 IAV isolated from a rosy-billed pochard (WT557/H6N2). After 20 passages in chickens, the variant 20Ch557/H6N2 virus replicated more efficiently in chickens than the parental WT557/H6N2 strain (Figure 1). Overall, not only higher peak virus titers were observed but also the number of chickens and days with positive tracheal and cloacal swabs was higher in chickens inoculated with the 20Ch557/H6N2 virus compared to those inoculated with the WT557/H6N2 strain (Figure 1). Animals infected with 20Ch557/H6N2 showed virus shedding from trachea and cloaca from day 1 post-infection (dpi) till 7 dpi, while chickens inoculated with WT557/H6N2 shed virus from trachea up to 5 dpi and showed limited replication in cloaca (only 1 bird) up to 3 dpi (no virus shedding was detected in cloaca by 5 dpi or thereafter). Out of 10 chickens infected with the SAm H6 IAVs, virus shedding through the trachea was observed in all chickens infected with 20Ch557/H6N2 and only in five chickens infected with WT557/H6N2 at 1 and 3 dpi (p < 0.05), confirming better fitness of this virus in comparison to parental WT557/H6N2. Virus titers in trachea from 20Ch557/H6N2 inoculated chickens were significantly higher than WT557/H6N2 inoculated chickens at 1 and 3 dpi (Figure 1A, p = 0.0155 and p = 0.0093, respectively). Also, virus titers in cloaca from 20Ch557/H6N2 inoculated chickens were significantly higher than WT557/H6N2 inoculated chickens at 1 dpi (Figure 1B, p = 0.0372). Although overt signs of disease were detected in neither group of the inoculated chickens, the results indicate that the chicken-adapted virus replicates better than WT557/H6N2 in trachea and cloaca, increasing viral shedding and for a longer time post-infection; thus, our laboratory adaptation strategy has increased viral fitness of the duck origin SAm H6N2 virus in chickens.
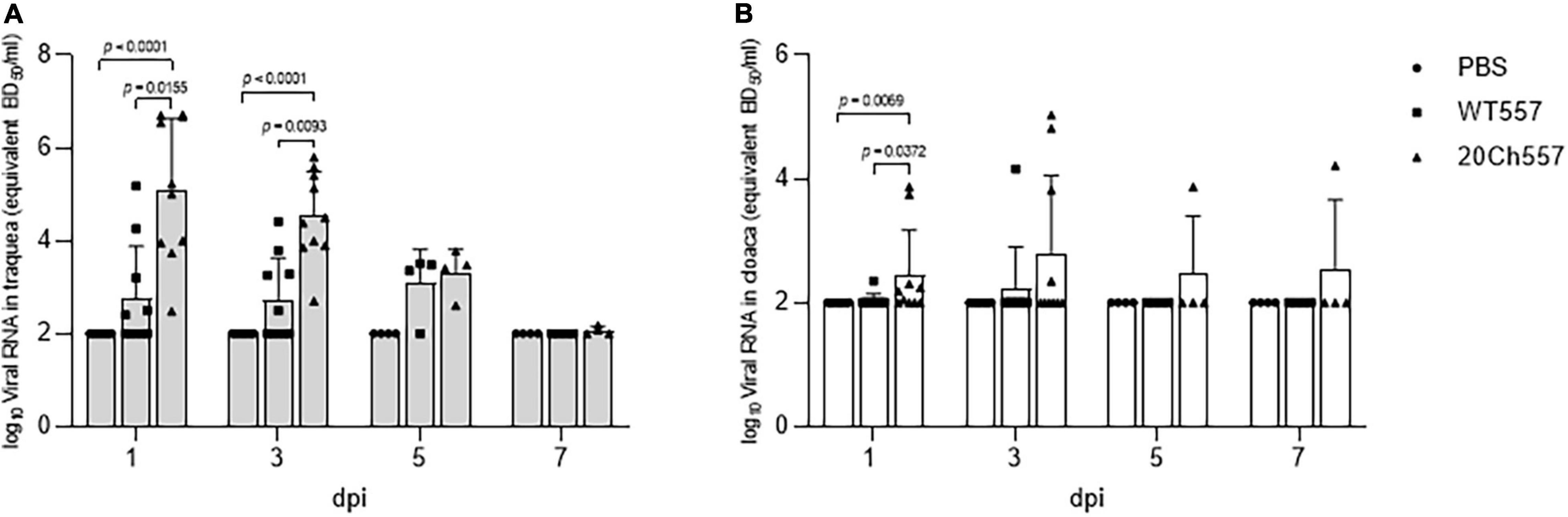
Figure 1. Replication of wild type and chicken-adapted H6N2 viruses in trachea and cloaca of chickens. Tracheal (A) and cloacal (B) swabs from control and IAV-infected chickens were analyzed for the presence of viral RNA (equivalent EID50/ml) at different days post-infection (dpi). For statistical purposes, tracheal and cloacal swabs without viral RNA detection were given a numeric value of 102 EID50/ml, which represents the lowest detectable level of viral RNA with the RT-qPCR used. Graphs show the mean and standard deviation (SD). p-values were determined using ANOVA with multiple testing (Kruskall–Wallis test and Dunn’s Test).
Differential Replication of WT557/H6N2 and 20Ch557/H6N2 Viruses in the Tissues of Chickens
To better determine the extent of virus replication of WT557/H6N2 and 20Ch557/H6N2 strains, a subset of chickens from each inoculated group (n = 6/group) was sacrificed at 3 dpi and bursa of Fabricius, lung, and intestinal tissues were collected. Mean bursa-to-body weight ratios were reduced in both IAV-inoculated groups in comparison to the negative control group (Figure 2, p = 0.0179 and p = 0.0039 for WT557/H6N2 and 20Ch557/H6N2 group, respectively), consistent with IAV infection in chickens. However, no significant differences in bursa-to-body weight ratios were observed between challenge viruses. All lung samples (n = 6) from the 20Ch557/H6N2 inoculated group was RNA virus positive, in contrast to a single lung sample (n = 1 out 6) from WT557/H6N2 inoculated group (p < 0.05) (Table 1). In addition, virus titers detected in lungs from the 20Ch557/H6N2 group were significantly higher compared to those from the WT557/H6N2 group (p < 0.05). No significant differences were observed in either the number of positive samples or in levels of virus titers in intestinal samples for either IAV inoculated group. This is also consistent with the PCR results, in which significantly higher replication was observed in tracheal swabs, and only a slightly higher replication was observed in cloacal swabs. These results are consistent with the strategy of virus adaptation that selects for strains with improved respiratory tropism. In this case, such improvement does not appear to alter the natural tropism for the intestinal tract typical of wild aquatic bird origin IAVs.
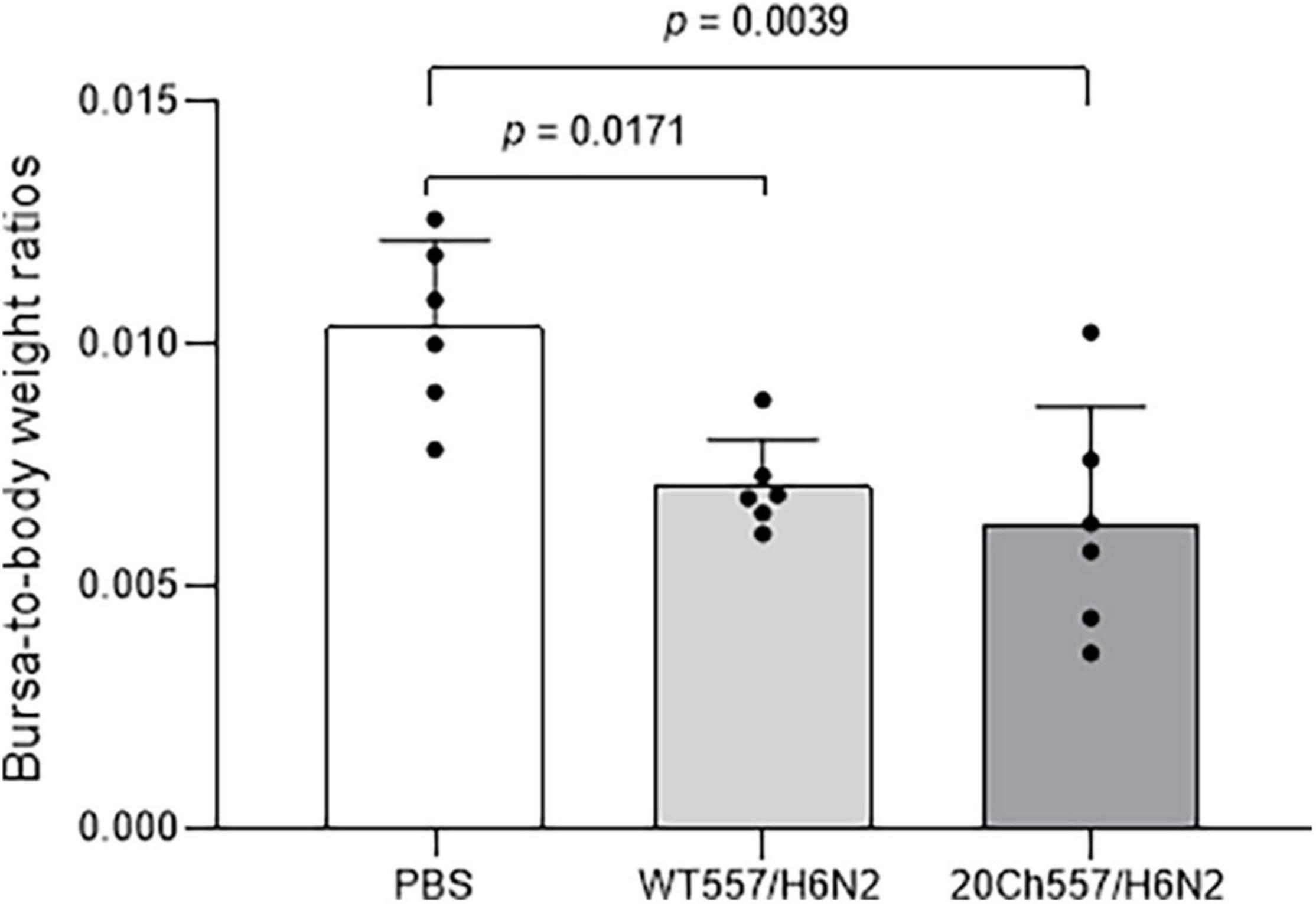
Figure 2. Tissue alteration after infection with wild type and chicken-adapted H6N2 viruses. Alterations of bursa-to-body weight ratios the following infection with WT557/H6N2 or 20Ch557/H6N2 virus, 3 dpi. Mean values for IAV-infected and control animals were compared. The results are expressed as the mean (SD) from each group, n = 6. p-values were determined using ANOVA with multiple testing (Tukey’s Test).
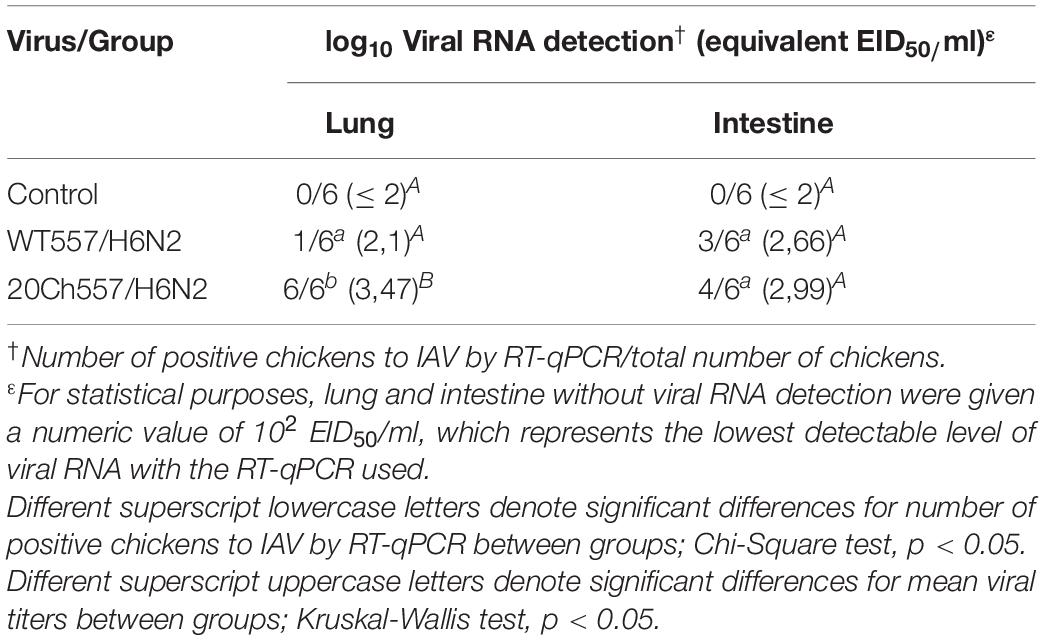
Table 1. Replication of wild type and chicken-adapted H6N2 viruses in lung and intestine of infected chickens.
Then, to determine whether SAm wild type or chicken-adapted H6N2 virus induces differences in the recruitment of mononuclear cells and/or lymphocyte subpopulations, the percentages of different lymphoid subsets in ileum from IAV-inoculated chickens were compared to those from control animals. Lamina propria and intraepithelial lymphocytic (IEL) cell compartments from the ileum were analyzed. Remarkably, in both compartments, there was an increase in total mononuclear cells in response to the infection, being significantly higher only in the case of 20Ch557/H6N2 infected animals (Figure 3A, p < 0.05). Furthermore, an increase in the percentages of CD3+TCRγδ+ lymphocytes in the ileum of chickens inoculated with 20Ch557/H6N2 virus was observed (Figure 3B, p < 0.05), being in part due to an increase in CD3+TCRγδ+ CD8a+ subpopulation. Besides, the macrophage population positive for staining of KUL01 marker showed a trend to increase in proportion in lamina propria from ileum from 20Ch557/H6N2 chickens (Figure 3C), as described during LPAIV infection (Abdul-Cader et al., 2018). The percentages of other subsets analyzed from ileum did not show differences between groups (data not shown). These results indicate that 20Ch557/H6N2 not only replicates better than WT557/H6N2 in the respiratory tract of chickens but also induces a higher cellular immune recruitment in the ileum.
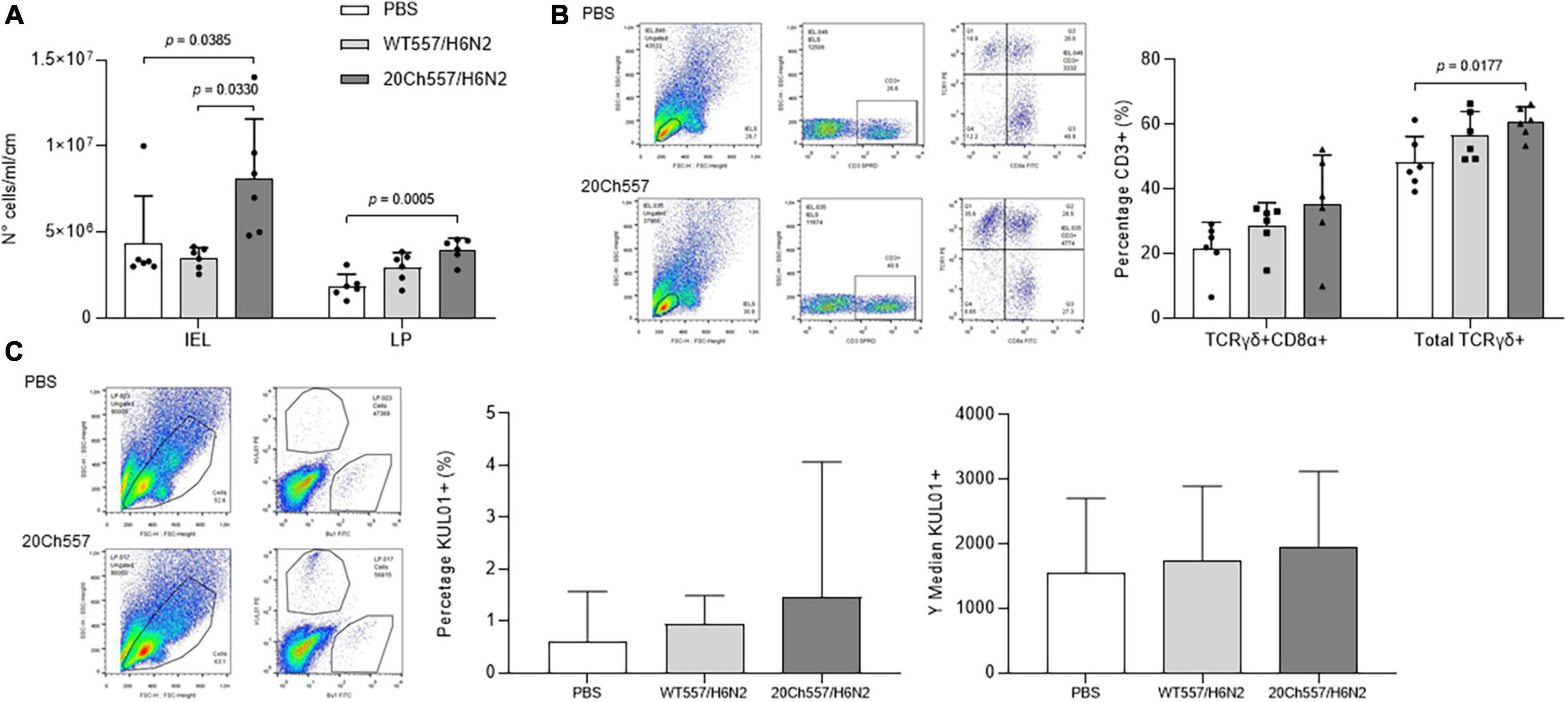
Figure 3. Differences in the recruitment of mononuclear cells and/or lymphocyte subpopulations in ileum of IAV-infected chickens. Six animals per group were euthanized at 3 dpi after infection with IAV or inoculation with PBS at 3 weeks of age. Ileum was collected and lamina propria and epithelial compartments were processed for flow cytometry analyses. (A) Mononuclear cell recovery from epithelial (IEL) and lamina propria (LP) compartment. Results are expressed as the mean (SD) of total amount of cells/ml recovered from each compartment from ten cm of ileum. p-values were determined using ANOVA with multiple testing (Dunn’s Test for IEL and Tukey’s Test for LP). (B) Chicken infection with 20Ch557/H6N2 virus increases TCRγδ+ IELs from ileum. Cells were labeled with anti-CD3, anti-CD8α and anti-TCRγδ antibodies conjugated to SPRD, FITC and PE, respectively. Analysis was performed in the gate of lymphocytes according to forward/side scatter parameters, selecting CD3+ cells followed by TCRγδ-PE/CD8α-FICT. The results obtained in epithelial compartment analysis are shown, expressed as the mean in percentage (SD) from each group. p-values were determined using ANOVA with multiple testing (Tukey’s Test). (C) Macrophages in lamina propria from ileum of chickens infected with 20Ch557/H6N2 virus. Cells were labeled with anti-KUL01 and anti-Bu1 antibodies conjugated to PE and FITC, respectively. Analysis was performed in the gate according to forward/side scatter parameters as shown followed by KUL01-PE/Bu1-FICT. The results obtained in lamina propria analysis are shown, expressed as the mean (SD) in percentage and MFI (SD) from KUL01+ population from each group.
Mutations Observed in the 20Ch557/H6N2 Virus Genome After Laboratory Adaptation of WT557/H6N2 Virus
To characterize the molecular features that allowed the chicken-adapted H6N2 virus to replicate more in the trachea, lung, and cloaca of chickens (and also to a longer extent in trachea and cloaca), we compared deduced amino acid sequences from all open reading frames of WT557/H6N2 and 20Ch557/H6N2 viruses.
Amino acid mutations were observed in the internal components of the chicken-adapted virus, either in structural or in non-structural proteins (Table 2). The only exceptions were NP and M2 proteins, which showed no amino acid substitutions. The PB2 protein of the 20Ch557/H6N2 virus showed one amino acid difference compared to WT557/H6N2 virus at position 748 (R748Q). Another single amino acid substitution was observed in the PB1 protein of the chicken-adapted H6N2 virus at position 484 (L484I). This mutation also produces the F83S change observed in the PB1-F2 protein of 20Ch557/H6N2 virus, which might be unique to the chicken-adapted virus since there are no PB1-F2 influenza sequences from SAm lineage in GenBank with this mutation. Then, there were two amino acid substitutions in the PA protein of 20Ch557/H6N2 virus, one at position 142 (K142R) – which is also encoded by PA-X protein of the adapted virus – and the other at position 336 (R336L). There were other two amino acid substitutions, one in M1 (L103I) and the other in NS1 (A86T) proteins of the 20Ch557/H6N2 virus.
We also found amino acid mutations in the superficial genes from the 20Ch557/H6N2 virus (Table 2). The HA protein of chicken-adapted H6N2 has four amino acid changes at positions 127 (V127I), 153 (Y153H), 182 (K182N), and 346 (L346I) in the HA2 region of the hemagglutinin. Finally, the NA protein of the 20Ch557/H6N2 virus has one mutation at position 400 (N400S).
Molecular Changes in the 20Ch557/H6N2 Virus Do Not Enhance Transmission in Chickens
To study direct contact transmission of WT557/H6N2 and 20Ch557/H6N2 viruses, four naïve chickens were placed together with infected birds at 1 dpi. Trachea and cloacal swabs were collected at different days post-contact (dpc) and sera collected at 20 dpc were tested by competitive enzyme-linked immunosorbent assay (ELISA) for specific antibodies against influenza A virus. Transmission studies indicated that the SAm wild type and chicken-adapted H6 viruses were not transmissible through direct contact. All swabs from direct contact animals were negative for the presence of viral RNA and blood samples did not show the presence of antibodies against the influenza virus (data not shown). When we analyzed serum samples from infected chickens, we observed 100% of infection with 20Ch557/H6N2 virus (4 out of 4 animals positive for ELISA) and 75% of infection with WT557/H6N2 virus (3 out of 4 animals positive for ELISA) (Table 3). These results show that the mutations obtained after serial lung passages of WT557/H6N2 virus in chickens improve virus infectivity, but do not enhance transmission in this host.
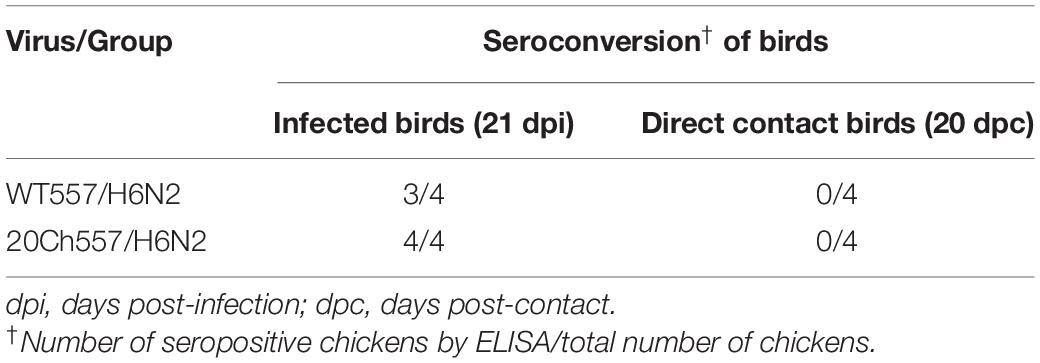
Table 3. Seroconversion of White Leghorn chickens against infection with wild type or chicken-adapted H6N2 virus.
Antigenic Relatedness Between WT557/H6N2 and 20Ch557/H6N2 Viruses
The antigenic properties of the SAm H6 viruses were investigated by using chicken antisera generated from the replication studies mentioned above. In general, antisera against the WT557/H6N2 and 20Ch557/H6N2 viruses showed low HI titers against the homologous virus (Table 4). It is notable that serum from chickens infected with 20Ch557/H6N2 showed lower HI titers against the homologous virus than against the heterologous virus. This result might be indicating that the antigenicity of the 20Ch557/H6N2 virus has been affected by the mutations introduced by the adaptation process, being the original WT557/H6N2 virus more immunogenic than the adapted one.
Discussion
Information about IAV found in the body of wild birds in South America is scarce (Hurt et al., 2014). However, we have clear evidence of the circulation of a fixed SAm internal gene constellation in IAV isolated from wild birds in Argentina since 2006 (Rimondi et al., 2018). Of the 60 IAV subtypes obtained to date – 22 IAVs determined have already been published, and among the 38 IAVs detected from 2017 to 2019, the predominant subtype observed is H6. Considering that Argentinean H6 IAVs could continue to evolve in wild birds, we conducted in vivo chicken studies with H6 IAV strain of South American lineage isolated from wild duck and its adapted version in chickens, thereby getting valuable and unknown knowledge on the pathogenesis and transmission of influenza A viruses isolated from wild birds in the region. Our results showed that, even though there was no evidence of clinical signs of disease compatible with IAV infection, both viruses infected chickens (observed mainly by seroconversion) but were not transmitted to other birds through direct contact. This, together with the significant bursa-to-body weight ratios reduction observed in both infected groups, demonstrated that WT557/H6N2 and 20Ch557/H6N2 viruses behave like LPAIV in chickens as observed with viruses from other lineages (Spackman et al., 2010; Daoust et al., 2011; Pantin-Jackwood et al., 2017; Lin et al., 2020). There is evidence similar to ours, where the low viral shedding by the cloaca observed after infection with LPAIV significantly reduces virus transmission among birds (Ruiz-Hernandez et al., 2016). In field conditions where animals might be immunosuppressed and exposed to secondary infections and/or adverse environmental conditions, lower doses of virus might be sufficient for IAV infection and transmission in poultry (Bertran et al., 2018). Other authors also discussed that transmission efficiency in experimental conditions is underestimated due to an artifact of our housing (isolators with high rates of airflow and grate floors) and the lack of concomitant management factors common in commercial farms (Pantin-Jackwood et al., 2017). Thus, we should not rule out the possibility that changes in clinical presentation and/or transmission in chickens infected with WT557/H6N2 or 20Ch557/H6N2 viruses could occur in the field when accompanied by concomitant factors, as demonstrated by other authors (Spickler et al., 2008; Bertran et al., 2018).
Importantly, avian influenza is an exotic disease in Argentina because there is no evidence of IAV circulation in commercial poultry to date. Consequently, vaccination against the influenza virus is not being implemented in the country. There have been several reports of H6 subtype IAV outbreaks in domestic poultry in Europe, North America, Asia, and Africa in recent decades (Hoffmann et al., 2000; Webby et al., 2002; Abolnik et al., 2007, 2019; Huang et al., 2010). Furthermore, a SAm H6 IAV was isolated in backyard chickens in Chilean Polynesia for the first time in 2019 (Di Pillo et al., 2022), and phylogenetic analysis of all gene segments revealed that the virus isolated was closely related to IAVs circulating in South America. Remarkably, the PB2, PB1, and NS gene segments are closely related to viruses isolated in Argentina. Taken together, the results we obtained after chicken infection with wild-type and adapted IAV from SAm lineage add valuable information to the scientific veterinary community about the possible introduction of H6 IAV in poultry in Argentina and its further adaptation in chicken, emphasizing the importance of implementation of good biosecurity and management practices to avoid the introduction of IAV in poultry industry from Argentina.
Sequencing data from WT557/H6N2 and 20Ch557/H6N2 viruses was important to correlate the mutations observed (after 20 passages in chickens) to the higher viral replication observed in the respiratory tract of chickens infected with 20Ch557/H6N2 virus (Figure 1 and Table 1), which enabled the viral tropism to shift from the exclusively gastrointestinal tract (known to occur mostly in wild ducks) to a combination of the respiratory and digestive tract (commonly observed in Galliformes) (Costa et al., 2012; Kuiken, 2013). However, it is difficult to know if all or just only some of these amino acid changes are responsible for the higher fitness observed in vivo. The mutation observed in the NS1 (A86T) protein of the 20Ch557/H6N2 virus has been already described in other influenza viruses, however, no clear association to species-specificity has been attributed to this variation (Rajsbaum et al., 2012; Nogales et al., 2017). Furthermore, no mammalian-associated virulence markers in PB2 (E627K and D701N) (Subbarao et al., 1993; Hatta, 2001; Shinya et al., 2004) or NS1 protein (T92E) (Heui Seo et al., 2002) were found in the mutations observed in the chicken-adapted H6N2 virus. Some authors studied the importance of four conserved PB1 motifs in influenza virus transcription/replication (Motif I to IV) (Chu et al., 2012). For this, motif IV should have isoleucine at position 484 in PB1, as we observed in the 20Ch557/H6N2 virus. This mutation also produces the F83S change observed in the PB1-F2 protein of 20Ch557/H6N2. Considering that the PB1-F2 ORF from 20Ch557/H6N2 virus is 90 amino acids in length – as commonly found in AIV isolated from ducks – and that the all PB1-F2 proteins from AIV from SAm lineage have phenylalanine at position 83 instead of serine, further functional analyses of the PB1-F2 protein from 20Ch557/H6N2 virus should be considered to determine its role in influenza pathogenicity in chickens.
With respect to the superficial genes, the HA protein of the 20Ch557/H6N2 virus differed from the WT557/H6N2 virus in the HA2 region at positions 127 (V127I), 153 (Y153H), 182 (K182N), and 346 (L346I). Interestingly, the three first amino acid substitutions in HA are part of the Receptor Binding Site (RBS). Functional studies are necessary to determine if these mutations increase the infective capacity of the adapted strain to chicken epithelial cells through better interaction with the sialic acid receptor. Considering that previous results showed that the interaction of recombinant HA proteins with sialic acid receptors may play a critical role in γδ T-cell activation (Lu et al., 2013), together with our observation of an increment of this cell subpopulation in the ileum of chickens infected with20Ch557/H6N2 virus, our results might be indicative of an important role in the mutations observed in the RBS of HA protein of the chicken-adapted strain. Furthermore, the mutation K182N incorporates a new glycosylation site in the RBS. Glycosylation in this region has been described as a mechanism used by influenza virus to escape from neutralizing antibody response (Altman et al., 2018; Doud et al., 2018). Thus, this modification could also change antigenicity of the RBS and explain the lower antibody response elicited by the 20Ch557/H6N2 virus (Table 4) in spite of showing overall higher titers and longer period of viral detection compared to the parental strain. In addition, Dong et al. (2018) showed that the glycosylation of HA plays a significant role in the activation of γδ T cells, suggesting that the increase in the percentage of CD3+TCRγδ+ lymphocytes observed in the epithelial cell compartment from our study could be related to the higher viral replication of 20Ch557/H6N2 virus and also to the acquisition of an additional glycosylation site in HA protein after chicken adaptation.
Then, the NA gene of the 20Ch557/H6N2 virus differed from the WT557/H6N2 virus at position 400 (N400S). This mutation in NA protein is not associated with drug resistance (McKimm-Breschkin, 2000; Abed et al., 2005). Interestingly, position 400 is in one of three loops that form a second sialic acid-binding site that is typical of bird-influenza virus (Du et al., 2019, 2020). Remarkably, the N in position 400 is mainly found in strains that infect different bird species outside the Galliformes family, whereas S in position 400, as found in our chicken-adapted strain, is mainly found in viral variants identified in isolates from chickens (Kobasa et al., 1997; Du et al., 2019). This might be indicative that the N400S mutation observed in the 20Ch557/H6N2 virus increases viral fitness in poultry. The available data of the mutations observed in IAV proteins, together with the results obtained from our chicken study, outlines the importance to determine which out of the thirteen amino acid changes found in the 20Ch557/H6N2 virus are responsible for the higher fitness observed in vivo. Also, it would be interesting having knowledge of the number of passages that are necessary for chickens for the appearance of the critical mutations that change pathogenesis in this host.
Note worthily, we could not discard the possibility that certain parameters of H6 IAVs, such as infectivity, pathogenicity, and/or transmission in chickens, might be different if we had used another H6 virus isolated in Argentina for the adaptation strategy. However, the H6 viruses that we have been detecting in this country do not acquire significant amino acid mutations along their genome to date (>98% sequence identity in the H6 segment), which might be indicative that these viruses are well adapted to duck species found in Argentina. More important, none of the H6 isolates showed the mutations we found through our adaptation strategy in chickens. In addition, it is difficult to ensure if more passages or recombination between IAVs would enable virus transmission and/or increase pathogenicity. Secondary infections, immunosuppression, or adverse environmental conditions commonly found under field conditions might enable the acquisition of other mutations, or even fasten the occurrence of the mutations here reported. Therefore, biosecurity and management practices in commercial and backyard flocks should be considered in Argentina.
In summary, our in vivo studies with IAV of SAm lineage showed that WT557/H6N2 and 20Ch557/H6N2 viruses replicated in SPF chickens but do not transmit by direct contact. The genetic modifications acquired by the 20Ch557/H6N2 virus provided greater fitness in terms of infection, replication, and excretion in SPF chickens, triggering a greater immune response in the intestinal epithelium of this host at least at 3 dpi but showing lower immunogenicity. Taken together, these results provide valuable information regarding the immunopathogenesis and transmission of avian influenza viruses from SAm lineage, which may be useful for future prevention strategies to improve the health status of poultry in the region. Finally, considering that IAVs belonging to the H5, H7, and H9 subtypes have been found circulating in wild birds from South America and that HPAIV outbreaks in poultry were reported in the region (Suarez et al., 2004; Spackman et al., 2006); our results highlight the importance of further exploring the pathogenicity and transmission in chickens of other subtypes of IAV of SAm lineage to determine their risk to poultry production.
Data Availability Statement
The datasets presented in this study can be found in online repositories. The names of the repository/repositories and accession number(s) can be found in the article/Supplementary Material.
Ethics Statement
The animal study was reviewed and approved by National Institute of Agricultural Technology Ethics Committee (INTA, Argentina).
Author Contributions
AR, MR, and DP: study design. AR and VO: virus sequencing. AR, VO, and IS: animal studies with sample and data collection. AR, GP, and MR: interpretation of protein mutations. AR, IS, and MR: data analysis and interpretation of flow cytometry. AR: writing of the manuscript. MR and DP: revision of the manuscript. All authors approved the manuscript before its submission.
Funding
The National Institute of Allergy and Infectious Diseases (NIAID) Center for Research on Influenza Pathogenesis (CRIP) (contracts HHSN266200700010C and HHSN272201400008C) and the Instituto Nacional de Tecnología Agropecuaria (INTA) (PNSA 1115052 and PNSA 1115056) funded this study. This work was also supported by CONICET, through a fellowship gave to Agustina Rimondi during her postdoc. The content is solely the responsibility of the authors and does not represent official views of the National Institutes of Health.
Conflict of Interest
The authors declare that the research was conducted in the absence of any commercial or financial relationships that could be construed as a potential conflict of interest.
Publisher’s Note
All claims expressed in this article are solely those of the authors and do not necessarily represent those of their affiliated organizations, or those of the publisher, the editors and the reviewers. Any product that may be evaluated in this article, or claim that may be made by its manufacturer, is not guaranteed or endorsed by the publisher.
Acknowledgments
The authors thank Mariela Gammella for her help with the animal studies. The National Institute of Allergy and Infectious Diseases (NIAID) Center for Research on Influenza Pathogenesis (CRIP) (contracts HHSN266200700010C and HHSN272201400008C) and the Instituto Nacional de Tecnología Agropecuaria (INTA) (PNSA 1115052 and PNSA 1115056) funded this study. This work was also supported by CONICET, through a fellowship given to Agustina Rimondi during her postdoc. The content is solely the responsibility of the authors and does not represent official views of the National Institutes of Health. The results presented in this manuscript have previously appeared online in a preprint version (Rimondi et al., 2022).
Supplementary Material
The Supplementary Material for this article can be found online at: https://www.frontiersin.org/articles/10.3389/fmicb.2022.953738/full#supplementary-material
References
Abdelwhab, E. S. M., Veits, J., and Mettenleiter, T. C. (2013). Genetic changes that accompanied shifts of low pathogenic avian influenza viruses toward higher pathogenicity in poultry. Virulence 4, 441–452. doi: 10.4161/viru.25710
Abdul-Cader, M. S., Ehiremen, G., Nagy, E., and Abdul-Careem, M. F. (2018). Low pathogenic avian influenza virus infection increases the staining intensity of KUL01+ cells including macrophages yet decrease of the staining intensity of KUL01+ cells using clodronate liposomes did not affect the viral genome loads in chickens. Vet. Immunol. Immunopathol. 198, 37–43. doi: 10.1016/j.vetimm.2018.02.009
Abed, Y., Goyette, N., and Boivin, G. (2005). Generation and Characterization of Recombinant Influenza A (H1N1) Viruses Harboring Amantadine Resistance Mutations. Antimicrob. Agents Chemother. 49, 556–559. doi: 10.1128/AAC.49.2.556-559.2005
Abolnik, C., Bisschop, S., Gerdes, T., Olivier, A., and Horner, R. (2007). Outbreaks of avian influenza H6N2 viruses in chickens arose by a reassortment of H6N8 and H9N2 ostrich viruses. Virus Genes 34, 37–45. doi: 10.1007/s11262-006-0007-6
Abolnik, C., Strydom, C., Rauff, D. L., Wandrag, D. B. R., and Petty, D. (2019). Continuing evolution of H6N2 influenza a virus in South African chickens and the implications for diagnosis and control. BMC Vet. Res. 15:455. doi: 10.1186/s12917-019-2210-4
Altman, M. O., Angeletti, D., and Yewdell, J. W. (2018). Antibody Immunodominance: The Key to Understanding Influenza Virus Antigenic Drift. Viral Immunol. 31, 142–149. doi: 10.1089/vim.2017.0129
Bertran, K., Lee, D. H., Criado, M. F., Smith, D., Swayne, D. E., and Pantin-Jackwood, M. J. (2018). Pathobiology of Tennessee 2017 H7N9 low and high pathogenicity avian influenza viruses in commercial broiler breeders and specific pathogen free layer chickens. Vet. Res. 49, 1–9. doi: 10.1186/s13567-018-0576-0
Bertran, K., Sá, E., Silva, M., Pantin-Jackwood, M. J., and Swayne, D. E. (2013). Protection against H7N3 high pathogenicity avian influenza in chickens immunized with a recombinant fowlpox and an inactivated avian influenza vaccines. Vaccine 31, 3572–3576. doi: 10.1016/j.vaccine.2013.05.039
Chu, C., Fan, S., Li, C., Macken, C., Kim, J. H., Hatta, M., et al. (2012). Functional analysis of conserved motifs in influenza virus PB1 protein. PLoS One 7:e36113. doi: 10.1371/journal.pone.0036113
Costa, T., Chaves, A. J., Valle, R., Darji, A., van Riel, D., Kuiken, T., et al. (2012). Distribution patterns of influenza virus receptors and viral attachment patterns in the respiratory and intestinal tracts of seven avian species. Vet. Res. 43:28. doi: 10.1186/1297-9716-43-28
Daoust, P. Y., Kibenge, F. S. B., Fouchier, R. A. M., van de Bildt, M. W. G., Van Riel, D., and Kuiken, T. (2011). Replication of low pathogenic avian influenza virus in naturally infected mallard ducks (Anas platyrhynchos) causes no morphologic lesions. J. Wildl. Dis. 47, 401–409. doi: 10.7589/0090-3558-47.2.401
Di Pillo, F., Baumberger, C., Salazar, C., Galdames, P., Ruiz, S., Sharp, B., et al. (2022). Novel Low Pathogenic Avian Influenza H6N1 in Backyard Chicken in Easter Island (Rapa Nui), Chilean Polynesia. Viruses 14:718. doi: 10.3390/v14040718
Dong, P., Ju, X., Yan, Y., Zhang, S., Cai, M., and Wang, H. (2018). γδ T Cells Provide Protective Function in Highly Pathogenic Avian H5N1 Influenza A Virus Infection. Front. Immunol. 9:2812. doi: 10.3389/fimmu.2018.02812
Doud, M. B., Lee, J. M., and Bloom, J. D. (2018). How single mutations affect viral escape from broad and narrow antibodies to H1 influenza hemagglutinin. Nat. Commun. 9, 1–12. doi: 10.1038/s41467-018-03665-3
Du, W., Guo, H., Nijman, V. S., Doedt, J., van der Vries, E., van der Lee, J., et al. (2019). The 2nd sialic acid-binding site of influenza a virus neuraminidase is an important determinant of the hemagglutinin-neuraminidase-receptor balance. PLoS Pathog. 15:e1007860. doi: 10.1371/journal.ppat.1007860
Du, W., Wolfert, M. A., Peeters, B., van Kuppeveld, F. J. M., Boons, G. J., de Vries, E., et al. (2020). Mutation of the second sialic acid-binding site of influenza A virus neuraminidase drives compensatory mutations in hemagglutinin. PLoS Pathog. 16:e1008816. doi: 10.1371/journal.ppat.1008816
Hatta, M. (2001). Molecular Basis for High Virulence of Hong Kong H5N1 Influenza A Viruses. Science 293, 1840–1842. doi: 10.1126/science.1062882
Heui Seo, S., Hoffmann, E., and Webster, R. G. (2002). Lethal H5N1 influenza viruses escape host anti-viral cytokine responses. Nat. Med. 8, 950–954. doi: 10.1038/nm757
Hoffmann, E., Stech, J., Guan, Y., Webster, R. G., and Perez, D. R. (2001). Universal primer set for the full-length amplification of all influenza A viruses. Arch. Virol. 146, 2275–2289. doi: 10.1007/s007050170002
Hoffmann, E., Stech, J., Leneva, I., Krauss, S., Scholtissek, C., Chin, P. S., et al. (2000). Characterization of the influenza A virus gene pool in avian species in southern China: was H6N1 a derivative or a precursor of H5N1? J. Virol. 74, 6309–6315. doi: 10.1128/jvi.74.14.6309-6315.2000
Hossain, M. J., Hickman, D., and Perez, D. R. (2008). Evidence of expanded host range and mammalian-associated genetic changes in a duck H9N2 influenza virus following adaptation in quail and chickens. PLoS One 3:e3170. doi: 10.1371/journal.pone.0003170
Huang, K., Bahl, J., Fan, X. H., Vijaykrishna, D., Cheung, C. L., Webby, R. J., et al. (2010). Establishment of an H6N2 influenza virus lineage in domestic ducks in southern China. J. Virol. 84, 6978–6986. doi: 10.1128/JVI.00256-10
Hurt, A. C., Vijaykrishna, D., Butler, J., Baas, C., Maurer-Stroh, S., Silva-de-la-Fuente, M. C., et al. (2014). Detection of Evolutionarily Distinct Avian Influenza A Viruses in Antarctica. mBio 5:e01098–14. doi: 10.1128/mBio.01098-14
Kobasa, D., Rodgers, M. E., Wells, K., and Kawaoka, Y. (1997). Neuraminidase hemadsorption activity, conserved in avian influenza A viruses, does not influence viral replication in ducks. J. Virol. 71, 6706–6713. doi: 10.1128/jvi.71.9.6706-6713.1997
Kuiken, T. (2013). Is low pathogenic avian influenza virus virulent for wild waterbirds? Proc. R. Soc. B Biol. Sci. 280:20130990. doi: 10.1098/rspb.2013.0990
Lin, W., Cui, H., Teng, Q., Li, L., Shi, Y., and Li, X. (2020). Evolution and pathogenicity of H6 avian influenza viruses isolated from Southern China during 2011 to 2017 in mice and chickens. Sci. Rep. 10, 1–12. doi: 10.1038/s41598-020-76541-0
Lu, Y., Li, Z., Ma, C., Wang, H., Zheng, J., Cui, L., et al. (2013). The interaction of influenza H5N1 viral hemagglutinin with sialic acid receptors leads to the activation of human γδ T cells. Cell. Mol. Immunol. 10, 463–470. doi: 10.1038/cmi.2013.26
McKimm-Breschkin, J. L. (2000). Resistance of influenza viruses to neuraminidase inhibitors–a review. Antivir. Res. 47, 1–17. doi: 10.1016/s0166-3542(00)00103-0
Nogales, A., Martinez-Sobrido, L., Topham, D. J., and DeDiego, M. L. (2017). NS1 Protein Amino Acid Changes D189N and V194I Affect Interferon Responses, Thermosensitivity, and Virulence of Circulating H3N2 Human Influenza A Viruses. J. Virol. 91:e01930–16. doi: 10.1128/jvi.01930-16
Olsen, B., Munster, V. J., Wallensten, A., Waldenström, J., Osterhaus, A. D. M. E., and Fouchier, R. A. M. (2006). Global patterns of influenza a virus in wild birds. Science 312, 384–388. doi: 10.1126/science.1122438
Pantin-Jackwood, M. J., Stephens, C. B., Bertran, K., Swayne, D. E., and Spackman, E. (2017). The pathogenesis of H7N8 low and highly pathogenic avian influenza viruses from the United States 2016 outbreak in chickens, turkeys and mallards. PLoS One 12:e0177265. doi: 10.1371/journal.pone.0177265
Pereda, A. J., Uhart, M., Perez, A. A., Zaccagnini, M. E., La Sala, L., Decarre, J., et al. (2008). Avian influenza virus isolated in wild waterfowl in Argentina: evidence of a potentially unique phylogenetic lineage in South America. Virology 378, 363–370. doi: 10.1016/j.virol.2008.06.010
Prager, F., Wei, D., and Rose, A. (2017). Total Economic Consequences of an Influenza Outbreak in the United States. Risk Anal. 37, 4–19. doi: 10.1111/risa.12625
Rajsbaum, R., Albrecht, R. A., Wang, M. K., Maharaj, N. P., Versteeg, G. A., Nistal-Villán, E., et al. (2012). Species-Specific Inhibition of RIG-I Ubiquitination and IFN Induction by the Influenza A Virus NS1 Protein. PLoS Pathog. 8:e1003059. doi: 10.1371/journal.ppat.1003059
Rimondi, A., Gonzalez-Reiche, A. S., Olivera, V. S., Decarre, J., Castresana, G. J., Romano, M., et al. (2018). Evidence of a fixed internal gene constellation in influenza A viruses isolated from wild birds in Argentina (2006–2016). Emerg. Microbes Infect. 7:194. doi: 10.1038/s41426-018-0190-2
Rimondi, A., Olivera, V. S., Soria, I., Parisi, G. D., Rumbo, M., and Perez, D. R. (2022). Few amino acid mutations in H6 influenza A virus from South American lineage increase pathogenicity in poultry. bioRxiv [Preprint]. doi: 10.1101/2022.04.28.489980
Rimondi, A., Xu, K., Craig, M. I., Shao, H., Ferreyra, H., Rago, M. V., et al. (2011). Phylogenetic analysis of H6 influenza viruses isolated from rosy-billed pochards (Netta peposaca) in Argentina reveals the presence of different HA gene clusters. J. Virol. 85, 13354–13362. doi: 10.1128/JVI.05946-11
Ruiz-Hernandez, R., Mwangi, W., Peroval, M., Sadeyen, J. R., Ascough, S., and Balkissoon, D. (2016). Host genetics determine susceptibility to avian influenza infection and transmission dynamics. Sci. Rep. 6, 1–11. doi: 10.1038/srep26787
Shinya, K., Hamm, S., Hatta, M., Ito, H., Ito, T., and Kawaoka, Y. (2004). PB2 amino acid at position 627 affects replicative efficiency, but not cell tropism, of Hong Kong H5N1 influenza A viruses in mice. Virology 320, 258–266. doi: 10.1016/j.virol.2003.11.030
Spackman, E., Gelb, J., Preskenis, L. A., Ladman, B. S., Pope, C. R., Pantin-Jackwood, M. J., et al. (2010). The pathogenesis of low pathogenicity H7 avian influenza viruses in chickens, ducks and turkeys. Virol. J. 7, 1–10. doi: 10.1186/1743-422X-7-331
Spackman, E., McCracken, K. G., Winker, K., and Swayne, D. E. (2006). H7N3 avian influenza virus found in a South American wild duck is related to the Chilean 2002 poultry outbreak, contains genes from equine and North American wild bird lineages, and is adapted to domestic turkeys. J. Virol. 80, 7760–7764. doi: 10.1128/JVI.00445-06
Spickler, A. R., Trampel, D. W., and Roth, J. A. (2008). The onset of virus shedding and clinical signs in chickens infected with high-pathogenicity and low-pathogenicity avian influenza viruses. Avian Pathol. 37, 555–577. doi: 10.1080/03079450802499118
Stringa, P., Papa-Gobbi, R., Vela, M., Gentilini, M. V., Machuca, M., Klin, P., et al. (2021). Native Spleen Preservation During Visceral Transplantation Inhibits Graft-Versus-Host-Disease Development: Clinical and Experimental Study. Ann. Surg. [Epub ahead of print]. doi: 10.1097/SLA.0000000000004979
Suarez, D. L., Senne, D. A., Banks, J., Brown, I. H., Essen, S. C., Lee, C. W., et al. (2004). Recombination resulting in virulence shift in avian influenza outbreak, Chile. Emerg. Infect. Dis. 10, 693–699. doi: 10.3201/eid1004.030396
Subbarao, E. K., London, W., and Murphy, B. R. (1993). A single amino acid in the PB2 gene of influenza A virus is a determinant of host range. J. Virol. 67, 1761–1764. doi: 10.1128/jvi.67.4.1761-1764.1993
Tong, S., Li, Y., Rivailler, P., Conrardy, C., Castillo, D. A., Chen, L. M., et al. (2012). A distinct lineage of influenza A virus from bats. Proc. Natl. Acad. Sci. U.S.A. 109, 4269–4274. doi: 10.1073/pnas.1116200109
Tong, S., Zhu, X., Li, Y., Shi, M., Zhang, J., Bourgeois, M., et al. (2013). New world bats harbor diverse influenza A viruses. PLoS Pathog. 9:e1003657. doi: 10.1371/journal.ppat.1003657
Webby, R. J., Woolcock, P. R., Krauss, S. L., and Webster, R. G. (2002). Reassortment and interspecies transmission of North American H6N2 influenza viruses. Virology 295, 44–53. doi: 10.1006/viro.2001.1341
Webster, R. G., Cox, N. J., and Sthor, K. (2002). WHO Manual on Animal Influenza Diagnosis and Surveillance. Geneva: World Health Organization. Available online at: http://www.who.int/csr/resources/publications/influenza/whocdscsrncs20025rev.pdf
Keywords: influenza A virus, South American IAV, viral adaptation, viral fitness, viral transmission, chicken studies, risk to poultry, immunopathogenesis
Citation: Rimondi A, Olivera VS, Soria I, Parisi GD, Rumbo M and Perez DR (2022) Few Amino Acid Mutations in H6 Influenza A Virus From South American Lineage Increase Viral Replication Efficiency in Poultry. Front. Microbiol. 13:953738. doi: 10.3389/fmicb.2022.953738
Received: 26 May 2022; Accepted: 21 June 2022;
Published: 27 July 2022.
Edited by:
Svetlana Khaiboullina, University of Nevada, Reno, United StatesReviewed by:
Shigefumi Okamoto, Kanazawa University, JapanKateri Bertran, IRTA-CReSA, Centre for Research on Animal Health, Spain
Copyright © 2022 Rimondi, Olivera, Soria, Parisi, Rumbo and Perez. This is an open-access article distributed under the terms of the Creative Commons Attribution License (CC BY). The use, distribution or reproduction in other forums is permitted, provided the original author(s) and the copyright owner(s) are credited and that the original publication in this journal is cited, in accordance with accepted academic practice. No use, distribution or reproduction is permitted which does not comply with these terms.
*Correspondence: Agustina Rimondi, rimondia@rki.de, rimondi.agustina@inta.gob.ar
†Present address: Agustina Rimondi, Division of Influenza Viruses and Other Respiratory Viruses, Robert Koch Institute, Berlin, Germany