- 1School of Energy and Environmental Engineering, Hebei University of Technology, Tianjin, China
- 2MOE Key Laboratory of Pollution Processes and Environmental Criteria, College of Environmental Science and Engineering, Nankai University, Tianjin, China
Trichloroethylene (TCE) is a ubiquitous chlorinated aliphatic hydrocarbon (CAH) in the environment, which is a Group 1 carcinogen with negative impacts on human health and ecosystems. Based on a series of recent advances, the environmental behavior and biodegradation process on TCE biodegradation need to be reviewed systematically. Four main biodegradation processes leading to TCE biodegradation by isolated bacteria and mixed cultures are anaerobic reductive dechlorination, anaerobic cometabolic reductive dichlorination, aerobic co-metabolism, and aerobic direct oxidation. More attention has been paid to the aerobic co-metabolism of TCE. Laboratory and field studies have demonstrated that bacterial isolates or mixed cultures containing Dehalococcoides or Dehalogenimonas can catalyze reductive dechlorination of TCE to ethene. The mechanisms, pathways, and enzymes of TCE biodegradation were reviewed, and the factors affecting the biodegradation process were discussed. Besides, the research progress on material-mediated enhanced biodegradation technologies of TCE through the combination of zero-valent iron (ZVI) or biochar with microorganisms was introduced. Furthermore, we reviewed the current research on TCE biodegradation in field applications, and finally provided the development prospects of TCE biodegradation based on the existing challenges. We hope that this review will provide guidance and specific recommendations for future studies on CAHs biodegradation in laboratory and field applications.
1. Introduction
Trichloroethylene (TCE) is a chlorinated aliphatic hydrocarbon (CAH) that belongs to a class of chlorinated organic solvents (Honetschlägerová et al., 2019). The physicochemical properties of TCE are listed in Table 1 (Pant and Pant, 2010). In 2011, the global consumption of TCE has risen sharply to 428,000 tons (Baskaran and Rajamanickam, 2019), and TCE production was about 250,000 tons in China (Huang et al., 2014). Annual production of TCE in the United States (United States) is about 130,000 metric tons. TCE is used widely in various commercial and industrial applications (Shukla et al., 2014) and in many household products (Suttinun et al., 2013). Due to the inappropriate handling, storage, disposal, and accidental release, TCE has been detected ubiquitously in soil and groundwater in many countries worldwide (Xing et al., 2022; Yamazaki et al., 2022), including the United States (Steffan et al., 1999; Lee et al., 2012; de Guzman et al., 2018), Canada (Perez-de-Mora et al., 2014), France (Dugat-Bony et al., 2012; Walaszek et al., 2021), and China (Kuo et al., 2004; Kao et al., 2016). TCE contamination is known to account for 22% of soil and groundwater at superfund sites in the USA (USEPA, 2013), and was found in 57% of National Priorities List sites in 2015 (ATSDR, 2011; de Guzman et al., 2018).
The migration and fate of TCE in environment depend on the physicochemical properties of TCE and the hydrogeological characteristics of sites (Honetschlägerová et al., 2019). TCE has high molecular weight and is labeled as dense non-aqueous phase liquid (DNAPL), it tends to migrate from the unsaturated zone into the underlying aquifer (Figure 1A). When TCE reaches the clay layer through the subsoil, some TCE may accumulated by subsurface solids (Figure 1B), or is transported through the clay layer (Honetschlägerová et al., 2019). The phases distribution of TCE in unsaturated zone is presented in Figure 1C. Furthermore, TCE may adsorbed by organic and mineral components in groundwater matrices, such as sediments and rocks, which may prevent partial TCE from migrating (Pant and Pant, 2010). TCE residues can be a major source of long-term contamination. As the groundwater flows, so does the contaminated water, creating a pollution plume. At the same time, TCE slowly dissolves into the groundwater, reaching the dissolution limit (Honetschlägerová et al., 2019). In this case, not only TCE is presented in the plume but also reduced products such as cis-1,2-dichloroethylene (cis-DCE), trans-1,2-dichloroethene (trans-DCE), 1,1-dichloroethene (DCE), and vinyl chloride (VC) exist (Honetschlägerová et al., 2019).
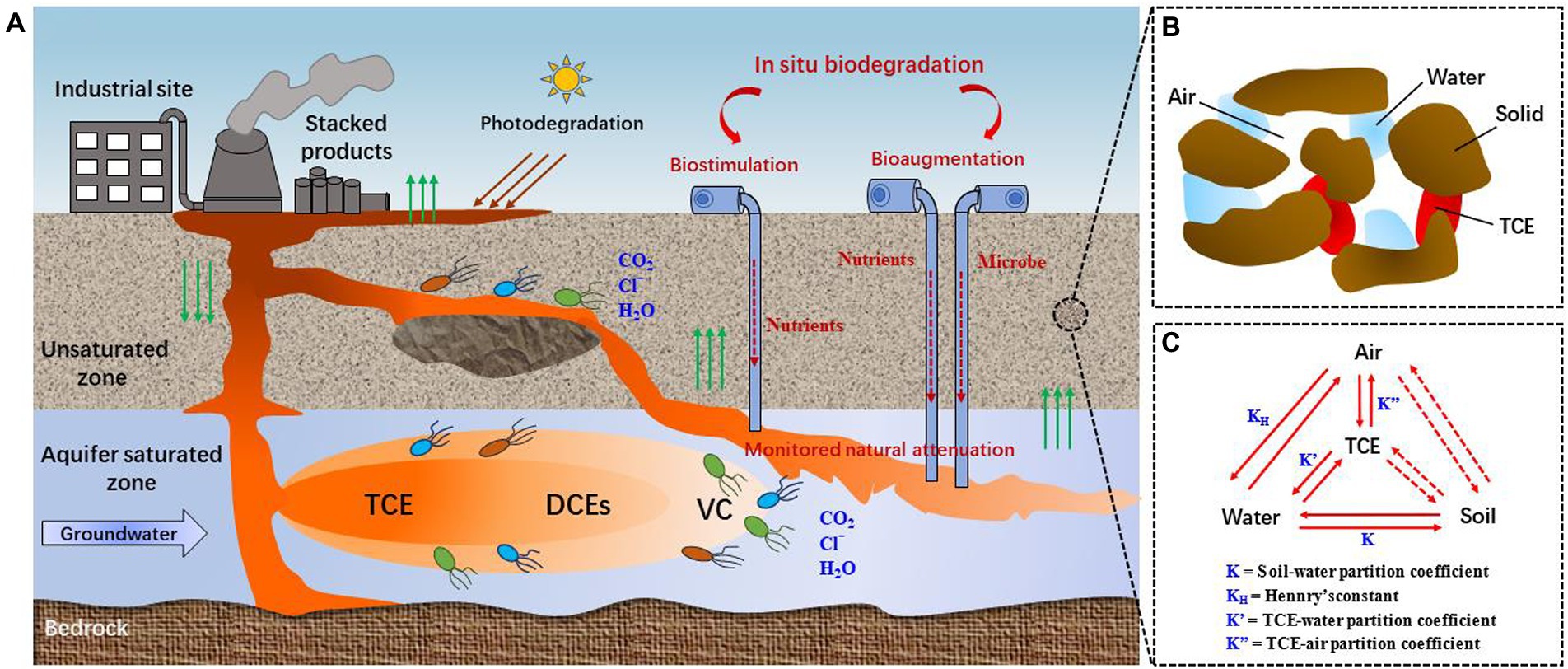
Figure 1. Migration and fate of TCE underground. (A) Conceptual model of the transportation of TCE underground [reproduced from (Xing et al., 2022)]; (B) Interactions of different phases of TCE; (C) Phase distribution of TCE in unsaturated zone.
TCE is a ubiquitous environmental contaminant, which has adverse effects on both ecosystem and human health (Tachachartvanich et al., 2018; Xiao et al., 2020). As a Group 1 carcinogen, TCE may increase the cancer risk of the kidney, cervix, liver and biliary passages, as well as non-Hodgkin lymphoma and esophageal adenocarcinoma (Johnson et al., 1998; Wartenberg et al., 2000; Hansen et al., 2013; Vlaanderen et al., 2013; Siggins et al., 2021). In addition, TCE has been reported to cause endocrine disrupting effects (Tachachartvanich et al., 2018). Concerns about the toxicity of TCE have driven its control. TCE has been listed as a priority pollutant by the Ministry of Ecology and Environment of China, the United States Environmental Protection Agency (USEPA), and the European Commission (Liu et al., 2021). The USEPA has set an upper limit for TCE concentration in drinking water at 5 μg/L (USEPA, 2009). In China, the Grade V criterion of TCE is 210 μg/L according to the National Standard for Groundwater Quality (GB/T14848-2017). The soil pollution control standard of TCE is 20 mg/kg according to the Soil Environmental Quality Risk Control Standard for soil contamination of development land (GB36600-2018).
Over the past 50 years, human efforts have been made to remediate TCE pollution (Suttinun et al., 2013). Biodegradation of TCE has received extensive attention from researchers due to its cost-effectiveness, environmental friendliness, and sustainability (Shukla et al., 2014; Wang et al., 2022). Biodegradation utilizes the catabolic capabilities of microorganisms with specific enzymatic activities to degrade TCE (Shukla et al., 2014). So far, many advances have been made in the field of biodegradation of chlorinated compounds (Bhatt et al., 2007; Dolinova et al., 2017), such as aerobic co-metabolism strategies of chlorinated solvents (Semprini, 1997) and aliphatic organochlorine degradation in subsurface environments (Koenig et al., 2015). However, only four reviews were published on TCE biodegradation in 1995–2022, including biodegradation of TCE (Pant and Pant, 2010; Shukla et al., 2014), molecular and cellular fundamentals of TCE aerobic co-metabolism (Arp et al., 2001), and co-metabolism of TCE (Suttinun et al., 2013). There has been a gradual increase in the number of studies on TCE biodegradation in recent years (Islam et al., 2021; Liu et al., 2021; Qiu et al., 2022; Underwood et al., 2022), but no recent review on this topic has been published since 2014. Therefore, the environmental behavior and biodegradation process of TCE need to be reviewed systematically.
Here, an effort has been made to shed light on recent studies on TCE biodegradation. The specific objectives of this review are: (1) to review four main biodegradation processes that lead to TCE biodegradation by bacterial isolates and mixed culture: anaerobic reductive dechlorination, anaerobic cometabolic reductive dichlorination, aerobic co-metabolism, and aerobic direct oxidation; (2) to discuss the mechanisms, pathways, enzymes, and influence factors involved in TCE biodegradation; (3) to summarize the material-mediated enhanced TCE biodegradation technology; (4) to review research progress of in situ biodegradation of TCE, and to propose the future perspectives of TCE biodegradation.
2. Effects of TCE on bacterial community
Soil microbes are the first to encounter chemicals entering the soil environment, which are sensitive indicators of soil contamination because they can rapidly respond to ecosystem disturbances (Nemir et al., 2010; Wu et al., 2019; Li et al., 2019b; Zhou et al., 2020, 2021). Microbial community structure can be dramatically impacted at about 1 ppm TCE concentration in soil (Nemir et al., 2010). Besides, TCE concentration above 10 mg/kg has been demonstrated can reduce soil quality, affect soil microbial biomass, and inhibit soil organic matter decomposition and mineral cycling (Li et al., 2019b). Furthermore, the downward movement of TCE along with soil depth affects the microbial ecology in different soil layers, and subsoil microbial diversity and functions were reduced compared to the topsoil (Koner et al., 2022). So far, only two articles have reported the effect of TCE concentration on soil microbial community, and there are few studies on the effects of different TCE concentrations on microbial communities in different types of soil.
In general, the entry of TCE into soil or groundwater ecosystem alters environmental quality, disrupts the natural balance of microbial communities, and affects microbial density and abundance, community structure, and metabolic activity (Fries et al., 1997; Nemir et al., 2010; Koner et al., 2022). Microbial density returned to baseline after subsequent phenol-TCE treatment in a field study, but the original species richness was not recovered until after toluene-TCE treatment (Fries et al., 1997). Additionally, TCE can change the composition of soil bacteria, fungi and actinomycetes in Mollisol, including Acidobacteria, Proteobacteria, Planctomycetes, Chytridiomycota, Streptomycetales, Pseudonocardiales, Propionibacteriales, and Rhizobiales, thereby affecting soil carbon and nitrogen cycling processes and energy metabolism (Li et al., 2019b). Their subsequent study manifested that TCE pollution had a significant effect on nitrogen transformation (Li et al., 2020). Nevertheless, the underlying mechanism of the effects of TCE on soil microbial community structure and function remains unclear.
TCE can influence the community structure and activity of methanotrophs, while the relative abundance of Methylobacter increased with TCE level and exposure time, suggesting that Methylobacter is resistant to TCE and plays a leading function in TCE degradation (Kong et al., 2014). Moreover, microorganisms with functions of TCE degradation have been reported, such as Flavobacterium, Clostridium, Desulfotomaculum, Desulfuromonas, Nitrospira, Sphingomonas, Acidovorax, Bacillus, and Pseudomonas, indicating the adaptability of native bacteria to TCE pollution (Koner et al., 2022). Thus, microorganisms can develop multiple physiological tolerance mechanisms and metabolic strategies under stressful conditions to degrade diverse environmental pollutants in ecosystems (Zhou et al., 2020, 2022; Koner et al., 2022). Studies have shown that many TCE-degrading microorganisms existed in TCE-contaminated sites (Lowe et al., 2002; de Guzman et al., 2018; Gafni et al., 2020). Reductive dechlorination bacteria have been proved to be closely related to the TCE-dechlorinating degradation in TCE-contaminated superfund sites (Lowe et al., 2002; de Guzman et al., 2018). Moreover, local microbial communities were reported can oxidize TCE in polluted water from Israeli Coastal Aquifer (Gafni et al., 2020). A recent study showed that the degree of soil contamination by TCE was positively correlated with the abundance of TCE-degrading taxa (Koner et al., 2022). Therefore, functional bacteria in TCE-contaminated environments are important microbial resources for TCE biodegradation.
3. Biodegradation of TCE
Biodegradation is one of the most promising technologies for TCE degradation in soil and groundwater, which involves four major processes: (1) anaerobic reductive dechlorination, an anaerobic process in which TCE is the electron acceptor, and hydrogen and organic substrate are as the electron donor (Pant and Pant, 2010; Tiehm and Schmidt, 2011); (2) anaerobic cometabolic reductive dichlorination, TCE can be cometabolized in the presence of growth-supporting electron acceptors (DCEs and VC; He et al., 2003; Tang et al., 2013; Clark et al., 2018); (3) aerobic co-metabolism, requiring oxygen for enzymatic degradation of TCE, and yields no good for related bacteria (Pant and Pant, 2010); (4) aerobic direct oxidation, aerobic bacteria can utilize TCE as the only carbon source, and the produced water and carbon dioxide are non-toxic to other residential microorganisms (Koner et al., 2022). The characteristics of the four main processes are shown in Table 2.
3.1. Anaerobic reductive dechlorination
3.1.1. Bacterial isolates and mixed culture
In the anaerobic organohalide respiration, TCE acts as an electron acceptor, while chlorine is simultaneously removed from TCE (Ebrahimbabaie and Pichtel, 2021), and the energy from exergonic dehalogenation is utilized for microbial growth (Dolinova et al., 2017). In general, hydrogen and a variety of hydrogen-releasing substrates can be used as primary electron donors. The substrates are single compound substrates such as acetate, lactate, formate, pyruvate, benzoate, butyrate, methanol, ethanol, glucose, and propionate (Dolinova et al., 2017; Robles et al., 2021; Chen and Wu, 2022; Tomita et al., 2022; or complex substrates such as emulsified vegetable oil, soybean oil, surfactants, molasses, whey, and flour (Lee et al., 2012; Sheu et al., 2015; Dolinova et al., 2017; Chen and Wu, 2022; Dutta et al., 2022).
Bacterial isolates and mixed culture involved in anaerobic reductive dechlorination of TCE were summarized in Table 3, and a phylogenetic tree was constructed with the identified bacterial isolates (Figure 2A). As for bacterial isolates, organohalide-respiring bacteria (OHRB) have been confirmed to be highly effective TCE degrading strains (Fincker and Spormann, 2017), including species from Dehalococcoides (Maymo-Gatell et al., 1997; Maymó-Gatell et al., 1999; Gushgari-Doyle and Alvarez-Cohen, 2020; Asai et al., 2022) and Candidatus Dehalogenimonas (Chen et al., 2022), which can dechlorinate TCE to benign ethene. Besides, strains from Dehalobacter (Holliger et al., 1998; Rupakula et al., 2015), Enterobacter (Kang et al., 2012), Clostridium (Lo et al., 2020; Lin et al., 2021), and Acidimicrobiaceae (Ge et al., 2019) were also observed to effectively dechlorinate TCE (Table 3). In addition, immobilization technique has been used to alleviate the toxic effect of TCE on cells (Lo et al., 2020), and the advantages including: (1) cells are well protected from adverse conditions, which can be easily recovered from bulk solutions; (2) cells can maintain high density for long time; (3) fixed cell can alleviate the inhibitory effects of high TCE concentrations to cells by avoiding delay times (Chen et al., 2007; Yang et al., 2019; Lo et al., 2020).
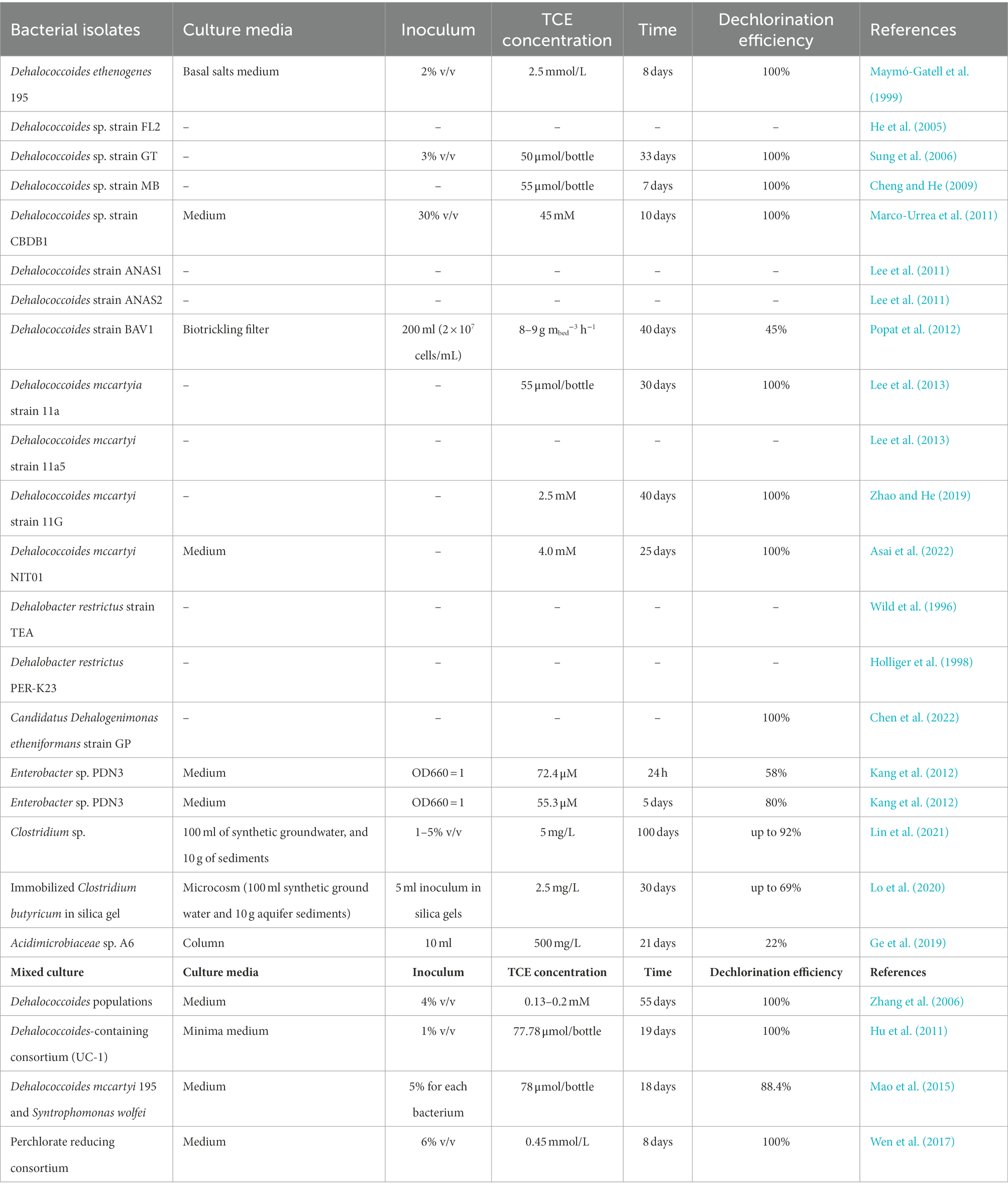
Table 3. Summary of anaerobic reductive dechlorination of TCE by bacterial isolates and mixed culture.
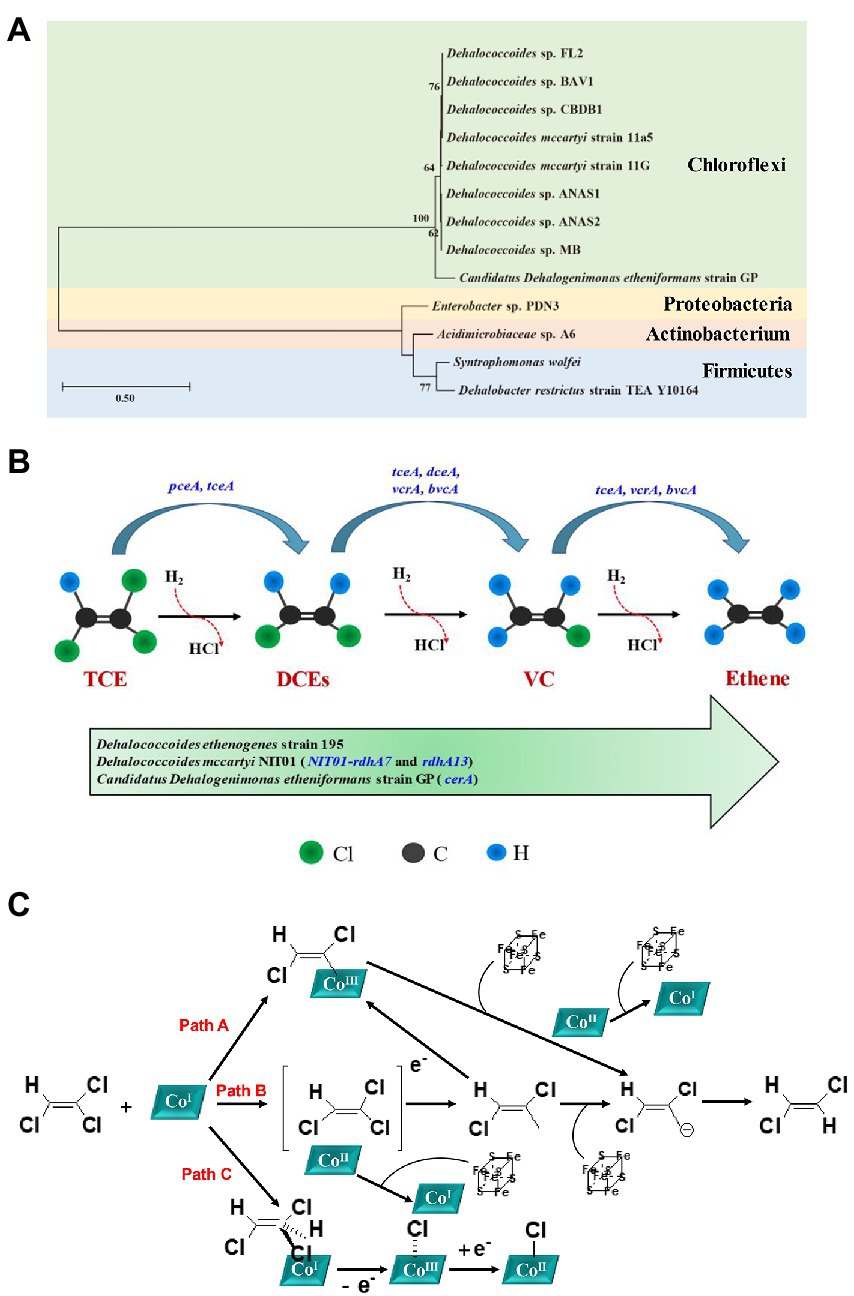
Figure 2. (A) Phylogenetic tree constructed based on the 16S rRNA sequences of bacterial isolates for anaerobic degradation of TCE; (B) Mechanism of completely anaerobic reductive dechlorination of TCE to ethene [adapted from (Maymo-Gatell et al., 1997; Dolinova et al., 2017; Lihl et al., 2019)]; (C) Proposed three reaction mechanisms of RDase: an organocobalt adduct (Path A), a single-electron transfer (Path B), and a halogen-cobalt bond (Path C; reproduced from (Jugder et al., 2015; Kunze et al., 2017).
Regarding to mixed culture, Dehalococcoides-containing cultures have received more attention because they can effectively reduce TCE to ethene (Table 3; Freeborn et al., 2005; Zhang et al., 2006; Hu et al., 2011). TCE can be completely biodegraded through spatiotemporal changes in several dehalorespiring species, including Sulfurospirillum, Dehalobacter, Desulfitobacterium, Geobacter, and Dehalococcoides (Dugat-Bony et al., 2012). Furthermore, a novel perchlorate-reducing dechlorinating bacteria has been manifested can reduce TCE up to 0.45 mmol/L to nontoxic ethene within 8 days after two additions of TCE (Wen et al., 2017).
3.1.2. Degradation mechanism, pathway, and enzyme
During reductive dechlorination, TCE acts as an electron acceptor in anaerobic microorganisms, linking the reductive dehalogenation of TCE with the synthesis of ATP through the electron transport chain (Wohlfarth and Diekert, 1997). Anaerobic microorganisms proliferate using H2 or organic substrates as electron donors, resulting in sequential substitution of chlorine atoms to produce lower chlorinated compounds (including cis-DCE, trans-DCE, VC), and eventually form ethene (Figure 2B; Dutta et al., 2022).
Reductive dehalogenase (RDase) is a key enzyme in OHRB such as Dehalococcoides, Dehalobacter, and is up-regulated during anaerobic reductive dechlorination (Zhang et al., 2020; Asai et al., 2022; Chen et al., 2022; Koner et al., 2022). RDase is a membrane-associated iron–sulfur protein containing an activated super nucleophilic form of the coenzyme vitamin B12, cob(I)alamin, which cleaves carbon-halogen bonds, and dechlorinates TCE via electroreduction (Zhang et al., 2020; Yan et al., 2021). The proposed three reaction mechanisms of RDase were presented in Figure 2C. The three reaction mechanisms were: (1) Path A: formation of a transient organocobalt adduct; (2) Path B: a single-electron transfer with CoI being the initial electron donor; and (3) Path C: halogen-cobalt bond formation. Among these three mechanisms, Path B is a long-distance electron transfer in outer-sphere, which suggested that electrons were transferred from CoI leading to substrate radical formation and finally to the formation of a carbanion after elimination of the halogen substituent. However, the internal mechanism as outer-sphere or inner-sphere route is still under debate. For example, Zhang et al. demonstrated that the B12-catalyzed reductive dechlorination of olefins in microbes should proceed through an inner-sphere electron transfer rather than outer-sphere electron transfer pathway (Zhang et al., 2021). Therefore, more efforts should be devoted to exploring the actual mechanisms of anaerobic reductive dechlorination. Overall, pceA, tceA (TCE to DCE), tceA, dceA, vcrA, bvcA (cis-DCE to VC), and tceA, vcrA, bvcA (VC to ethene) are crucial RDases involved in TCE reductive dechlorination process (Figure 2B; Ise et al., 2011; Popat and Deshusses, 2011; Dugat-Bony et al., 2012; Zinder, 2016; Dolinova et al., 2017; Mao et al., 2019; Yan et al., 2021; Rossi et al., 2022b). Furthermore, the recently discovered new strain Dehalococcoides mccartyi NIT01 contains 19 rdhA genes (including NIT01-rdhA7 and rdhA13), which are basically the same as the encoded vcrA and pceA (Asai et al., 2022). Moreover, Candidatus Dehalogenimonas etheniformans strain GP can combine formate and H2 oxidation with the reduction of TCE, DCE isomers, and VC to benign ethene using acetate as the carbon source, and the cerA gene plays a crucial role (Chen et al., 2022).
3.1.3. Influence factors
The reductive dechlorination of TCE is affected by biological aspects, physicochemical factors (e.g., pH, temperature, oxygen), and coexisting pollutants.
Co-culture of Dehalococcoides mccartyi 195 and Syntrophomonas wolfei can promote TCE degradation through efficient metabolic exchange and electron transfer as the result of interspecies aggregation during the syntrophic growth (Mao et al., 2015). The addition of Shewanella oneidensis MR-1 has been shown to have a positive effect on reductive dehalogenase activity and vitamin B12 uptake in cultures containing Dehalococcoides, which largely facilitated the complete dechlorination of TCE to ethene (Li et al., 2019c).
pH is a crucial factor affecting the growth of microbes (Yadvika et al., 2004). In an upflow anaerobic sludge blanket (UASB) reactor, TCE-degrading anaerobic granular sludge could effectively treated 36.5 mg/L TCE wastewater (80% removal rate) at pH 6.0–8.0 (Zhang et al., 2015a). Weakly acidic environment (pH < 7.0) has a greater influence on TCE removal (Zhang et al., 2015a). Temperature also plays a vital role in affecting the bioavailability of hydrophobic contaminants and influencing bacterial capability of growth and metabolism and enzymatic activities (Wang et al., 2022; Yamazaki et al., 2022). Temperature can influence the anaerobic degradation rate and products of TCE by a Dehalococcoides-containing consortium (UC-1), because it affects the acclimation period and leads to the selection of Dehalococcoides populations (Hu et al., 2013). Recently, Yamazaki et al. pointed out that the optimal temperature for TCE dechlorination in contaminated soil and groundwater is 25–35°C, and microbial community was notably impacted at 35°C (Yamazaki et al., 2022). In addition, oxygen can affect the dechlorination activity and change the overall biotransformation rate. The degradation of TCE by Dehalococcoides mccarty was prolonged when the oxygen concentration changed from 0 to 7.2 mg/L (Liu et al., 2017). Furthermore, hydraulic retention time affects the bacterial community structure, and TCE, as a recalcitrant compound, requires longer detention time for complete biodegradation. It was reported that the removal efficiency of TCE decreased from 99 to 85% when the hydraulic retention time is reduced from 25 to 5 h in a laboratory UASB reactor (Zhang et al., 2015b).
Many studies have shown that coexisting pollutants [e.g., chloroform, acetylene, perfluoroalkyl substances (PFASs), perfluoroalkyl acids (PFAAs), arsenic, perchlorate, and sulfate] can inhibit the biodegradation of TCE. Generally, the effects of these pollutants on the biodegradation of TCE are highly concentration dependent. Chloroform may affect dechlorinating organisms or may indirectly influence dechlorination by inhibiting other microorganisms (Duhamel et al., 2002). Chloroform in concentrations as low as 2.5 μM has been reported to inhibit TCE dechlorination by Dehalococcoides isolates and mixed cultures (Maymó-Gatell et al., 2001; Duhamel et al., 2002; Ding et al., 2020). Besides, acetylene influences a variety of microbial processes and typically inhibits redox-active metalloenzymes, which has been shown to be a potential inhibitor of a mixed anaerobic dehalogenation culture during TCE reductive dechlorination (Pon et al., 2003). The inhibition of acetylene on microbial processes is strongly dependent on its concentration. High concentration of acetylene (1.3 mM) reversibly inhibited TCE reductive dechlorination by Dehalococcoides mccartyi isolates and mixed cultures (Mao et al., 2017a). Fermentable components of Aqueous Film-Forming Foams (AFFFs) stimulated the dechlorination of TCE by microbial communities including Dehalococcoides mccartyi, while dechlorination could be inhibited by AFFF-derived perfluoroalkyl substances (PFASs; Harding-Marjanovic et al., 2016). Furthermore, the reductive dechlorination of TCE by a methanogenic mixed culture was significantly inhibited when exposed to perfluoroalkyl acids (PFAAs; 11 PFAA analytes, 6 mg/L each, totaling 66 mg/L; Weathers et al., 2016). Moreover, As(III) at a concentration of 9.1 μM reduced the cell growth of Dehalococcoides mccartyi by 50%, and affected the dechlorination activity of TCE (Gushgari-Doyle and Alvarez-Cohen, 2020). When the As(V) concentration reached 200 μM, there was no effect on TCE dechlorination at the initial stage, but inhibition was observed in cultures amended with 200 μM As(V) and 100 μM As(V) in 12 and 17 days, respectively, corresponding with the accumulation of As(III). Perchlorate preferentially uses electron donors compared to TCE, especially when electron donor is insufficient (Wen et al., 2016). Increasing perchlorate from 0 to 600 mg/L significantly decreased the relative abundance of TCE dechlorination bacteria Dehalococcoides (Wen et al., 2016). High sulfate concentration (5 mM) inhibited the reductive dechlorination of TCE by microbial communities containing Dehalococcoides due to the toxicity of the produced sulfide (Mao et al., 2017b).
3.2. Anaerobic cometabolic reductive dechlorination
Anaerobic cometabolic reductive dechlorination has also been described as a mechanism for anaerobic biodegradation of TCE (He et al., 2003; Tang et al., 2013; Clark et al., 2018). TCE can be co-metabolized in the presence of growth-supporting electron acceptors (He et al., 2003).
Cometabolic reductive dechlorination of TCE was first discovered in methanogenic cultures (Bouwer and McCarty, 1983; Vogel and McCarty, 1985). Methanogens and other bacteria have abundant reduced transition-metal cofactors that stochastically dechlorinate TCE under anaerobic conditions (Gantzer and Wackett, 1991). Unfortunately, the cometabolic reductive dechlorination rate of TCE decreases by an order of magnitude with each chlorine substituent removed, resulting in the accumulation of cis-DCE and VC (Gantzer and Wackett, 1991; Löffler et al., 2013). Dehalococcoides mccartyi strains play significant roles in catalyzing the reductive dechlorination of TCE to benign ethene under anoxic conditions (Magnuson et al., 2000; Loeffler et al., 2013; Clark et al., 2018). Notably, bvcA-carrying Dehalococcoides mccartyi strain BAV1 cannot grow with TCE, but can dechlorinate TCE to ethene when growth-supporting DCEs or VC are available (He et al., 2003; Krajmalnik-Brown et al., 2004; Löffler et al., 2013; Tang et al., 2013).
3.3. Aerobic co-metabolism
3.3.1. Bacterial isolates and mixed culture
Aerobic co-metabolism is a significant and widely studied biodegradation process of TCE. TCE co-metabolism was first demonstrated by Wilson and Wilson that TCE can be aerobically co-metabolized to CO2 in unsaturated soil columns exposed to a mixture of natural gas in air (0.6%; Wilson and Wilson, 1985). Aerobic co-metabolic biodegradation of TCE can be achieved through the supplement of primary carbon sources (Arcangeli and Arvin, 1997), including ammonia; some aliphatic compounds, such as methane, ethane, propane, propene, butane, and isoprene; and aromatics, such as toluene, creosol, and phenol (Chang and Alvarez-Cohen, 1995; Chen et al., 2007; Shukla et al., 2014; Yang et al., 2019; Gafni et al., 2020; Table 4). Among these substrates, toluene is reported to be the most efficient and practicable substrate (Hunt et al., 1995; Arcangeli and Arvin, 1997).
Bacterial isolates and mixed cultures that can aerobic co-metabolic degradation of TCE were displayed in Table 4. The bacterial phylogenetic tree was constructed based on the 16S rRNA sequences of isolated TCE biodegradation bacteria (Figure 3A). Most Pseudomonas sp. strains exhibit strong resistance to organic solvents, and some of them can aerobically co-metabolize TCE in the presence of toluene and phenol (Winter et al., 1989; Landa et al., 1994; Sun and Wood, 1996; Applegate et al., 1997; Ryoo et al., 2001; Chen et al., 2007; Chee, 2011; Abu Hamed et al., 2013; Li et al., 2014, 2015). Moreover, other strains from Mycobacterium (Wackett et al., 1989; Vanderberg et al., 1995), Burkholderia (Mars et al., 1996; Chee, 2011), Comamonas (Futamata et al., 2001; Zalesak et al., 2017), Methylosinus (Tsien et al., 1989), Methylomonas (Hanada et al., 1998), Alcaligenes (Harker and Kim, 1990), Xanthobacter (Reij et al., 1995), and Cupriavidus (Chang et al., 2021) were also effective TCE co-metabolizing bacteria (Table 4). Immobilized strain has also been proved to be an important method for promoting co-metabolism of TCE (Chen et al., 2007; Yang et al., 2019).
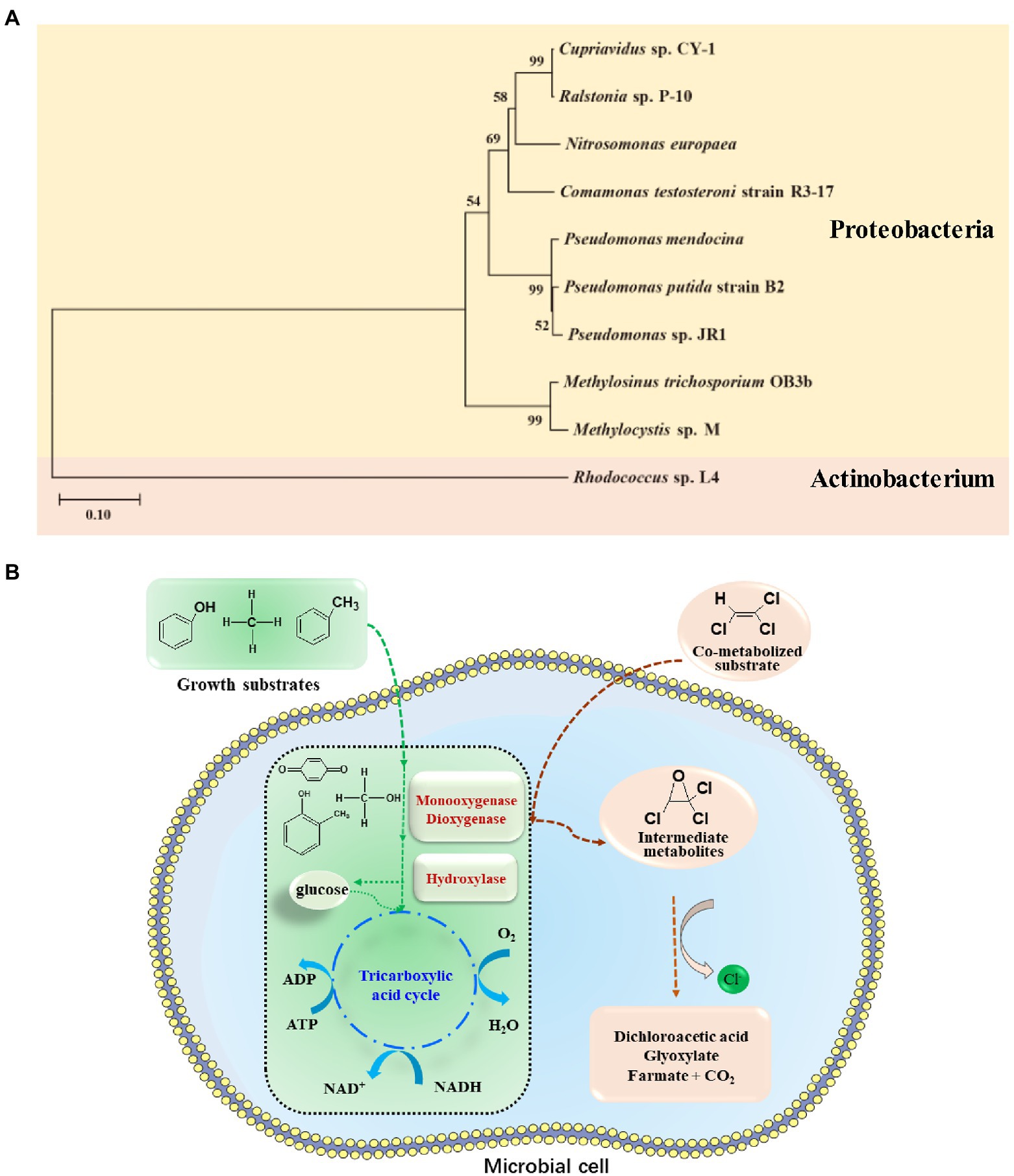
Figure 3. (A) Phylogenetic tree constructed based on the 16S rRNA sequences of isolated bacteria for TCE aerobic co-metabolism degradation; (B) Conceptual diagram of mechanism of aerobic co-metabolism of TCE with toluene, methane, and phenol.
As for mixed cultures, methane oxidizing bacteria (Wilson and Wilson, 1985; Fogel et al., 1986; Hanada et al., 1998), toluene-oxidizing bacteria (Han et al., 2007), phenol-degrading Variovorax strains (Futamata et al., 2005), as well as consortium bacteria including species from Pseudomonas, Mycobacterium, Methylocystis, Methylosinus, Nocardia, Nitrosomonas, Bordetella, Burkholderia, Ralstonia, and Comamonas have also been demonstrated to be important mixed cultures for TCE aerobic co-metabolism (Table 4; Sun and Wood, 1996; Hanada et al., 1998; Meza et al., 2003; Cutright and Meza, 2007; Zalesak et al., 2021).
Based on the above-described bacterial isolates and mixed cultures, single-stage, dual-stage, and three-stage reactors were designed for co-metabolize of TCE. Single-stage reactor systems of biofilm and immobilized strains were proposed for the co-metabolic degradation of TCE (Fitch et al., 1996; Arcangeli and Arvin, 1997; Shimomura et al., 1997; Yang et al., 2019). Besides, dual-stage reactor system was adopted to provide primary substrate and oxygen for cell growth in one compartment and send cells to the substrate-free compartment to provide TCE to avoid competitive inhibition during TCE degradation (Tschantz et al., 1995). In a two-stage bioreactor operating in single-pass and crossflow, >78 and 93% TCE degradation rates were achieved for 20 and 10 mg/L of TCE by Methylosinus trichosporium OB3b (Tschantz et al., 1995). Furthermore, a laboratory-scale three-stage rotating biological contactor was used to treat TCE-containing synthetic wastewater with a mixed biofilm populations including nitrifiers, heterotrophs, and Thiosphaera pantotropha, which achieved almost 100% removal rate when the TCE load was 0.0039 m3/m2 day (Brar and Gupta, 2000).
3.3.2. Degradation mechanism, pathway, and enzyme
Co-metabolism or co-metabolic degradation typically describes the ability of a microorganism to convert a non-growth supporting substrate (co-metabolized substrate or co-substrate) in the presence of a growth supporting substrate (primary substrate; Suttinun et al., 2013). Thus, the primary substrate supports microbial growth, while TCE does not enter the catabolic and anabolic pathways of microbial cell (Figure 3B). There are three reasons to elucidate why co-substrates such as TCE do not support microbial growth (Suttinun et al., 2013): (1) initiating enzymes transform substrates to products that are not further converted by other enzymes in the microorganism to generate metabolic intermediates for biosynthesis and energy production; (2) the initial substrate is converted to products that hinder subsequent enzyme activity during mineralization or inhibit bacterial growth; and (3) microorganisms need different substrates to trigger specific responses.
During aerobic co-metabolism, TCE binds to the enzymatic active site of various physiological substrates and is oxidized. As shown in Table 5, TCE can be oxidized by oxygenase generating microorganisms with broad auxiliary substrates, including methane, toluene, butane, ammonia, etc. Oxidation of TCE by monooxygenase, dioxygenase, or hydroxylase can lead to the formation of TCE epoxides, which are unstable and can be nonenzymatically degraded to various products, including glyoxylate, dichloroacetate, formate, and carbon dioxide (Figure 3B; Arp, 1995). Typically, methane oxidizing bacteria (methanotrophs) can use methane as sole carbon source, and co-metabolize TCE through nonspecific methane monooxygenase (MMO) enzymes (Shukla et al., 2009). In this process, MMO catalyzes the transformation of methane to methanol, and ultimately co-metabolizes TCE. Two genetically unrelated MMOs have been found and observed to be regulated by copper concentration (Shukla et al., 2009; Semrau et al., 2010), including soluble MMO (sMMO) expressed only by a subset of methanotrophs and ubiquitous membrane-bound particulate MMO (pMMO). The sMMO has the advantage of degrading TCE (Shukla et al., 2009), and purified pMMO can mineralize TCE to CO2 (Lontoh et al., 2000). Regarding toluene oxidizers, toluene monooxygenase and dioxygenase are efficient functional genes for TCE degradation, widely distributed in Pseudomonas (Table 5). In addition, isopropyl benzene dioxygenase (Dabrock et al., 1992; Pflugmacher et al., 1996), butane monooxygenase (Halsey et al., 2005), alkene monooxygenase (Ensign et al., 1992; Saeki et al., 1999), ammonia monooxygenase (Arciero et al., 1989; Rasche et al., 1991; Kocamemi and Cecen, 2010), phenol hydroxylase, and dichlorophenol hydroxylase (Harker and Kim, 1990) play crucial roles in the co-metabolic degradation of TCE (Table 5).
3.3.3. Influence factors
Studies have demonstrated that salinity, organic loading rate, toluene concentration, surfactant, and metal ion concentration are important influence factors affecting the aerobic co-metabolism of TCE. NaCl is a noncompetitive inhibitor for the degradation of toluene and TCE. As the instantaneous salinity increased from 0 to 3.5%, the maximum degradation rate of TCE by toluene-oxidizing cultures decreased from 2.28 to 1.45/day (Lee and Liu, 2006). In a methanogenic-methanotrophic coupled reactor, TCE degradation was influenced by organic loading rates, and possibly mediated by the effect of organic loading rate on methane (Lyew and Guiot, 2003). Increasing of organic loading rate was associated with increased dissolved methane level, which will lead to increased competition with TCE for the MMO and decreased TCE degradation (Lyew and Guiot, 2003). In a continuously fed biofilm reactor, TCE degradation by a toluene-oxidizing biofilm was strongly inhibited when toluene concentration exceeded 1 mg/L (Arcangeli and Arvin, 1997). Furthermore, biodegradable surfactants (Simple Green™ and soya lecithin) and primary substrate (cane molasses) can be used by indigenous microorganisms to improve the biodegradation efficiency of TCE during the aerobic co-metabolic process (Liang et al., 2011). Notably, metal ion concentrations have been shown to affect the co-metabolic biodegradation of TCE. Copper concentration can regulate the relative expression of pMMO, which has been proved to be a significant factor in the oxidation of TCE by Methylobacter sp. strain BB5.1 (Smith et al., 1997). Furthermore, 20 mg/L iron or 1 mg/L nickel enhanced the degradation of toluene and TCE by immobilized Pseudomonas putida F1 (Yang et al., 2019). This may be due to nickel and iron can provide necessary cofactors for the enzyme and improve the removal efficiency. A recent study found that the addition of low concentrations of zinc and copper facilitated the enzymatic conversion process and bioremediation of TCE in water by Pseudomonas plecoglossicida (Qiu et al., 2022).
3.4. Aerobic direct oxidation
Aerobic direct oxidation of TCE is a promising biological technology because it does not require auxiliary substrates and all available oxygen can be directly used for biodegradation (Gaza et al., 2019; Xing et al., 2022). In direct oxidation, microorganisms acquire organic carbon and energy by oxidatively degrading TCE (Pant and Pant, 2010). Mineralization of total TCE by aerobic microorganisms requires 2 moles of oxygen per mole of TCE, as shown in Eq. (1; Sun et al., 1998). As shown in Eq. (2), the oxidative biodegradation of TCE releases CO2 and H2O as end products (Baskaran and Rajamanickam, 2019).
Bacterial isolates and mixed cultures using TCE as the sole growth substrate were summarized in Table 6. The phylogenetic tree of the bacterial isolates was presented in Figure 4A. Rhodococcus sp. strains Sm-1 and Rhodococcus rhodochrous were able to mineralize 85 and 89% of TCE (1.1 mg/L) within 14 days, respectively (Malachowsky et al., 1994). The mineralization efficiencies of TCE (75 μM) by Pseudomonas and Methylosinus ranged from 71 to 109% in 6 h (Sun and Wood, 1996). Both two studies illuminated that oxygenase expression was responsible for TCE biodegradation (Malachowsky et al., 1994; Sun and Wood, 1996). In addition, Stenotrophomonas maltophilia PM102 degraded 90% of TCE (1.28 g/L) in 48 h at pH 7 and 77% of TCE in 72 h at pH 5 (Mukherjee and Roy, 2012). In a batch reactor, the removal rate of TCE (300 mg/L) reached >90% by microorganisms from turkey litter compost, among which Pseudomonas guguanensis NR135725 was the predominant strain for degrading TCE to CO2 and H2O (Baskaran and Rajamanickam, 2019). The proposed metabolic pathway of TCE aerobic biodegradation by Pseudomonas guguanensis NR135725 is shown in Figure 4B. A bacterial culture SF has been shown to degrade up to 400 mM TCE with an optimal temperature at 22°C and pH 7 in fixed-bed reactors and in batch experiments (Gaza et al., 2019).
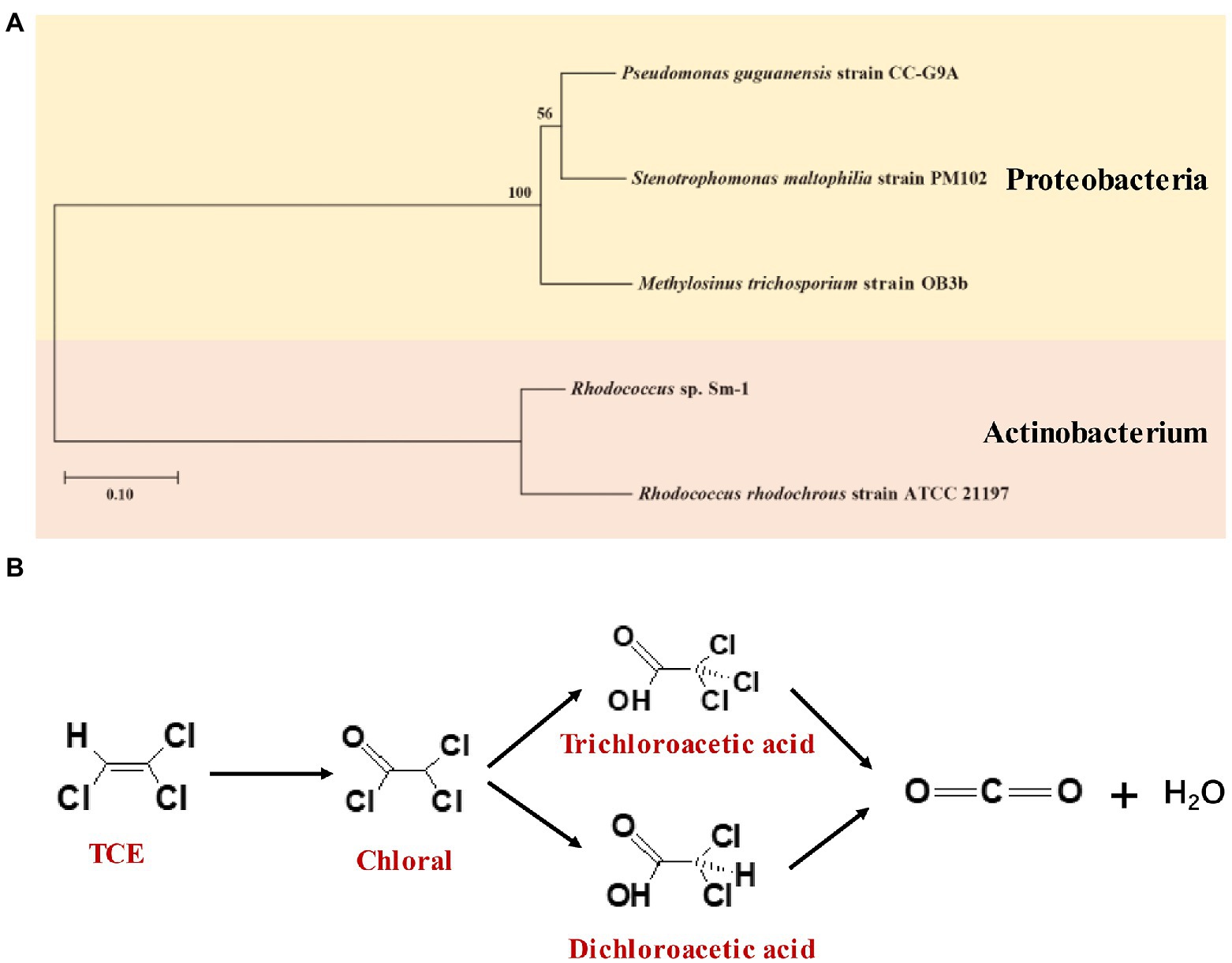
Figure 4. (A) Phylogenetic tree constructed based on the 16S rRNA sequences of isolated bacteria for TCE aerobic direct oxidation; (B) Aerobic direct oxidation of TCE by Pseudomonas guguanensis NR135725 [adapted from (Baskaran and Rajamanickam, 2019)].
Compared with anaerobic biodegradation and aerobic co-metabolism, the aerobic direct oxidation process of TCE has the advantage of no by-products. Therefore, it is necessary to isolate more aerobic bacteria for direct oxidation degradation of TCE in the future, and the mechanism of aerobic direct oxidation should be further studied.
3.5. Combined anaerobic and aerobic biodegradation
A combination of anaerobic (reduction) and aerobic (oxidation) is usually required to achieve TCE mineralization rather than partial conversion (Tartakovsky et al., 2003; Tresse et al., 2005; Dolinova et al., 2017). In particular, sequential anaerobic/aerobic biodegradation can conquer the shortcomings of using anaerobic and aerobic biodegradation alone (Bhatt et al., 2007; Tiehm and Schmidt, 2011; Frascari et al., 2013), and has the following main advantages: (1) prevents the accumulation of toxic metabolites; (2) does not require highly sensitive bacteria of the genus Dehalococcoides; (3) requires fewer electron donors as auxiliary substrates (Tiehm and Schmidt, 2011). So far, limited researches are available on the coupling of aerobic and anaerobic biodegradation of TCE (Tartakovsky et al., 2003; Tresse et al., 2005; Frascari et al., 2013; Polasko et al., 2019). A mutualistic consortium (especially methanotrophic and methanogenic microorganisms) almost completely degraded TCE at a loading of 18 mg/LR/day through the combination of reduction and oxidation pathways in an ethanol-fed biofilm reactor (Tartakovsky et al., 2003). Furthermore, TCE was completely degraded by different microorganisms using a three-step sequence of aerobic/anaerobic/aerobic treatment in batch bioreactors (Frascari et al., 2013).
4. Material-mediated enhanced biodegradation
Biodegradation of TCE takes a long time, and tends to result in the accumulation of regulated and more toxic transformation products, such as VC (Bruton et al., 2015). Therefore, more studies adopt enhanced biodegradation technology, especially material-mediated combined technologies to improve the biodegradation of TCE. From the existing researches, ZVI and biochar combined with microorganism have received more attention (Figure 5). Combined physicochemical and biological systems are more efficient for TCE degradation (Wang and Tseng, 2009; Wang et al., 2016).
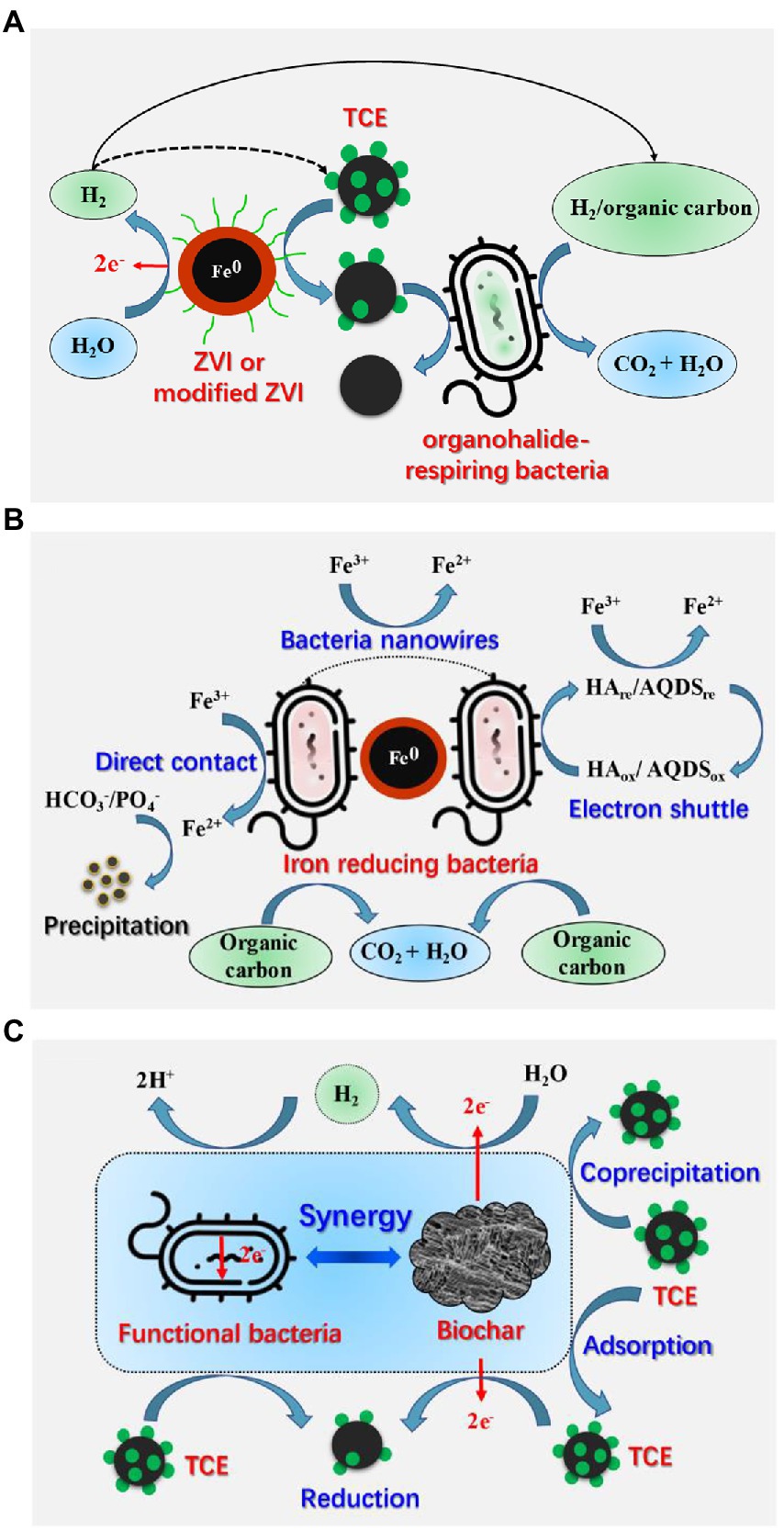
Figure 5. Mechanisms of material-mediated enhanced biodegradation of TCE. (A) The coupling mechanism of organohalide-respiring bacteria with ZVI or modified ZVI (reproduced from (Dong et al., 2019); (B) The coupling mechanism of iron reducing bacteria with ZVI [reproduced from (Dong et al., 2019)]; (C) The coupling mechanism of microorganisms with biochar.
4.1. ZVI-microorganism
Microbial reductive dechlorination combined with ZVI can improve TCE removal in several ways (Xiu et al., 2010; Tian and Yu, 2020; Wang et al., 2020): (1) ZVI can rapidly remove high concentration of TCE at early stage (Chen et al., 2012); (2) ZVI can reduce redox potential and enhance microbial activity of some functional bacteria (Chen et al., 2012; Wang et al., 2016, 2020); (3) H2 produced by ZVI is safer than the liquid hydrogen stored in steel tanks (Chen et al., 2012), which can be used as electron donor for anaerobic bacteria, including OHRB and iron reducing bacteria (IRB; Wang and Tseng, 2009; Xiu et al., 2010; Wang et al., 2016; Dong et al., 2019); (4) iron is an essential trace element for enzymes and catabolism and anabolism of microorganisms (Tian and Yu, 2020); (5) the low-valent iron Fe(II) and Fe(0) can be used as electron donors for microbes, while Fe(III) can be utilized as terminal electron acceptor for IRB such as Shewanella and Geobacter (Tian and Yu, 2020).
Many researches have been devoted to investigate the synergetic effects of ZVI, mZVI, nZVI, nZVI-CMC, FeS and functional microorganisms in promoting TCE removal (Figure 5A; Wang and Tseng, 2009; Kocur et al., 2016; Wang et al., 2016, 2020; Yang et al., 2018; Tian and Yu, 2020; Wu et al., 2020; Li et al., 2021; Yuan et al., 2022). The ZVI-bacteria combined system is not only a cost-effective technology, but also can greatly improve the TCE removal rate (Wang and Tseng, 2009). Sulfate reducing bacteria-ZVI system enables in situ sulfidation of ZVI, which offers a valuable strategy to overcome the limitations of biological or abiotic dechlorination degradation for TCE at sites (Islam et al., 2021). As for mZVI, the removal efficiency of TCE by mZVI combined with AHB was higher than that of pure mZVI, and particle sizes and dosages of the mZVI showed significant effects on the remediation performance of the mZVI-AHB system (Yuan et al., 2022). Furthermore, a field study suggested that mZVI combined with biostimulation can form a neutral, anoxic, and reducing condition in groundwater, which removed approximately 97.5% of chlorinated ethylene (including 12.8 and 14.23 μg/L TCE) and 80.2% of chlorinated ethane in 253 days (Wu et al., 2020). As for nZVI, in situ injection of nZVI stabilized with carboxymethyl cellulose (nZVI-CMC) significantly increased the functional bacterial populations (Dehalococcoides) and vinyl chloride reductase (vcrA) genes, thus promoting the complete and long-term dechlorination of chlorinated ethenes (Kocur et al., 2015, 2016). Moreover, co-encapsulated nZVI and Dehalococcoides species BAV1 system can degrade 10 mg/L of TCE within 3 h (Shanbhogue et al., 2017). Additionally, FeS has been demonstrated can enhance electron transfer for TCE dechlorination by Dehalococcoides mccartyi strain 195 (Li et al., 2021).
IRB can reduce Fe(III) to Fe(II) through multi-pathways and remove corrosion products on ZVI surface, thereby prolonging the service life of ZVI and improving the degradation efficiency of target pollutants (Figure 5B; Yang et al., 2017; Dong et al., 2019; Li et al., 2019a). For example, the combined system of ZVI and Shewanella alga BrY was able to degrade about 50% of TCE (30 mg/L) in 25 days (Shin et al., 2007), and the combination of Shewanella putrefaciens with aged mZVI can degrade 30 mg/L of TCE to 13 mg/L in about 20 days (Yang et al., 2017). Both studies revealed that Fe(II) ions generated by microbial reduction of Fe(III) could efficiently improve the TCE removal. However, nZVI may inhibit the removal rate of TCE by model IRB Shewanella algae CCM 4595, because the adsorption of cells to the passivated iron surface hinders electron transfer (Honetschlägerová et al., 2018).
4.2. Biochar-microorganism
Biochar-microorganism collaboration can promote the biodegradation of TCE (Figure 5C; Liu et al., 2021; Rossi et al., 2022b). The advantages include: (1) the adsorption of TCE on biochar can create a low-toxic environment for microorganisms; (2) the multi-layer porous structure of biochar can provide a habitat for microorganisms; (3) biochar can promote the biodegradation of TCE by stimulating the metabolic activity of functional anaerobic microorganisms (Aktaş et al., 2012; Liu et al., 2021). The biochar and landfill leachate microorganism packing column system can remove up to 99.7% of TCE (35 mg/L) removal, and the biochar prepared from waste material can support the formation of dechlorinated biofilms and facilitate the bioremediation of TCE (Siggins et al., 2021). Recently, commercial polyhydroxy butyrate combined with pine biochar improved the bioreductive dechlorination of TCE (approximately 80 mg/day) from aqueous solutions in a new reactor setup and small pilot scale (Rossi et al., 2022a). In a subsequent study, they confirmed that biofilm-biochar reactor was able to remove more than 99% of 100 μM TCE, and that Dehalococcoides mccartyi and tceA, bvcA, and vcrA genes played significant roles in TCE biodegradation (Rossi et al., 2022b). Furthermore, the addition of biochar supported nanoscale iron sulfide composite (CMC-FeS@biochar) followed by Corynebacterium variabile HRJ4 achieved 99% of TCE (10 mg/L) removal, and acetylene was the main product of the chemical process, whereas ethylene was the main product of the biological process (Lyu et al., 2018).
5. From laboratory scale studies to field application
According to the USEPA, bioremediation accounts for 24% of soil and groundwater remediation technologies (Wang et al., 2022). Compared with energy-intensive physical and chemical treatment methods, in situ bioremediation is an efficient method for TCE removal with less environmental impact and lower energy consumption (Rao et al., 2022; Rossi et al., 2022a; Yamazaki et al., 2022). Generally, three different approaches have been used widely for in situ bioremediation of TCE (Figure 1; Dolinova et al., 2017): (1) monitored natural attenuation (MNA), which uses natural abiotic and biotic degradation processes, and monitors the TCE plume over time to control the rate of natural attenuation and achieve site-specific remediation goals in time; (2) biostimulation, which combines the principle of MNA but involves the addition of carbon sources or electron donors to support and enhance TCE degradation by native microbial populations in contaminated environments; (3) bioaugmentation, which refers to the addition of pre-cultured degrading bacteria when the functional bacteria in the target environment are insufficient, and successful bioaugmentation often requires the addition of electron donors. Over the past few decades, numerous efforts have been dedicated to improve TCE biodegradation through biostimulation, bioaugmentation, and a combination of biostimulation and bioaugmentation (Löffler and Edwards, 2006; Polasko et al., 2019; Lo et al., 2020; Zalesak et al., 2021; Dutta et al., 2022; Underwood et al., 2022). It is worth noting that most of the field applications were conducted in the United States.
5.1. Biostimulation
The addition of single-compound (including phenol, toluene, nitrogen, phosphorus, methane, lactate) and complex substrates (such as slow polycolloid-releasing substrate, molasses, nZVI-CMC) has been shown to significantly promote the in situ TCE bioremediation. At the Moffett site (formerly the Moffett Naval Air Station), Mountain View, CA, phenol and toluene were used as the main substrates for the biodegradation of TCE, achieving 90% removal of 250 μg/L TCE in groundwater (Hopkins and McCarty, 1995). Delivery of vapor toluene with air promoted the growth of native toluene-utilizing bacteria that can degrade TCE through aerobic co-metabolism, and finally degraded TCE in aquifers to less than 10 mg/L (>90% removal; Kuo et al., 2004). Nitrogen and phosphorus addition has been observed can increase methanotroph populations and improve TCE biodegradation, which can influence at least 60 ft. above and to each side of the horizontal injection well at the Savannah River Area M bioremediation site, USA (Brockman et al., 1995). Besides, the addition of triethyl-phosphate and nitrous oxide to the pulsed injection of methane significantly stimulated in situ TCE degradation in groundwater at Westinghouse Savannah River Site in Aiken, South Carolina (Palumbo et al., 1995). A subsequent study at the same site confirmed that the injection of nutrients (methane, nitrogen, and phosphorus) facilitated the growth of TCE-degrading microorganisms and the biodegradation of TCE (Pfiffner et al., 1997). What’s more, injection of methane as an electron donor into the Snake River aquifer beneath the Test Area North site of the Idaho National Laboratory stimulated reductive dechlorination and co-metabolism of TCE by microorganisms in groundwater (Conrad et al., 2010). Complete biodegradation of TCE was achieved by lactate biostimulation of spatiotemporal changes in dehalorespiring bacterial community at a chlorinated solvent-contaminated site in France (Dugat-Bony et al., 2012).
For complex substrates, slow polycolloid-releasing substrate containing vegetable oil, cane molasses, and surfactants has been successfully adopted to remediate TCE contaminated groundwater in an industrial park site located in southern Taiwan (Tsai et al., 2014; Kao et al., 2016), and it removed up to 99% of TCE (1872 μg/L) in 50 days (Tsai et al., 2014). Another study in a TCE-contaminated groundwater site in Taiwan demonstrated that 97% of TCE was effectively degraded within 600 days with molasses injections (Liu et al., 2020). The above studies generally assumed that the existence of certain functional bacteria (e.g., Dehalococcoides, Dehalogenimonas, Pseudomonas, Sulfuricurvum) and genes (e.g., tceA, vcrA) were responsible for TCE biodegradation (Tsai et al., 2014; Kao et al., 2016; Liu et al., 2020). In addition, injection of nZVI-CMC stimulated natural OHRB and promoted dechlorination of chlorinated ethenes to nontoxic ethene at a field site in Sarnia, Ontario (Kocur et al., 2015, 2016). In the nZVI-CMC injection system, nZVI can generate hydrogen to create strong reducing conditions for bioreductive dechlorination, and at the same time, CMC is rapidly metabolized into cellulose, which is beneficial to in situ microbial reductive dechlorination (Kocur et al., 2015).
5.2. Bioaugmentation
Bioaugmentation refers to the direct injection of selected exogenous organisms into contaminated areas to facilitate the biodegradation of pollutants (Steffan et al., 1999). Methylosinus trichosporium OB3b and Burkholderia cepacian ENV435 have been manifested as two effective bacteria for field application (Duba et al., 1996; Steffan et al., 1999). Additionally, bioaugmentation using a mixed dechlorination culture KB-1 has been reported to significantly enhance in situ anaerobic bioremediation of TCE-contaminated groundwater (~13°C) in fractured carbonate rock at a site in Southern Ontario, Canada (Perez-de-Mora et al., 2014). Interestingly, a 3 to 4 orders of magnitude increase in the number of Bacteroidetes population in KB-1 was associated with an increase in ethene conversion (Perez-de-Mora et al., 2014). Recently, in a TCE-contaminated fractured rock aquifer, Trenton, N.J. in the USA, a bioaugmentation test with an emulsified vegetable oil solution (EOS®) and a dechlorinating consortium (KB-1®; containing Dehalococcoides) suggested that ethene levels were correlated strongly with Candidatus Colwellbacteria (p < 0.05), emphasizing the importance of functional bacterial populations for in situ biodegradation of TCE (Underwood et al., 2022).
5.3. Combination of biostimulation and bioaugmentation
So far, there are only two studies on the combination of biostimulation and bioaugmentation to degrade TCE. Dehalococcoides-containing cultures have been used in combined biostimulation and bioaugmentation systems. A pilot test at Launch Complex 34, Cape Canaveral Air Force Center indicated that native microbial communities can dechlorinate TCE to ethene when supplied with electron donors (methanol, ethanol, acetate, and lactate); however, bioaugmentation by a dechlorinating culture KB-1 markedly promoted the formation rate of ethene, and the removal rate of biological treatment was over 98.5% (Hood et al., 2008). They found that the abundance of Dehalococcoides increased by 2 orders of magnitude after biostimulation and bioaugmentation. A study at a TCE-contaminated groundwater site in Ft. Lewis, WA in the USA reported that biostimulation with whey (consisting of 10–13% of protein and 70–75% of lactose) and bioaugmentation with a Dehalococcoides-containing culture was an effective strategy to enhance TCE dechlorination by altering microbial populations (Lee et al., 2012).
6. Challenges and future perspectives
Based on the above review and discussion, several challenges and perspectives should be considered by future research:
Although some TCE-degrading microorganisms have been isolated and described, so far, the number of strains that can be applied in situ and completely dechlorinate TCE is very limited. For example, some effective Dehalococcoides strains are highly sensitive bacteria, which may restrict their use in field conditions. Therefore, it is necessary to isolate or discover more tolerant and efficient strains, such as facultative bacteria, to improve the efficient and complete biodegradation of TCE in laboratory and field applications. More importantly, compared with pure culture, the isolation and enrichment of highly efficient mixed cultures for TCE biodegradation is an important research direction.
In addition, the degradation intermediate products and metabolic mechanisms of TCE by different genera are different, and more TCE degradation pathways and genes need to be fully elucidated in the future. The coexistence of various microorganisms, such as the growth of other native bacteria (e.g., methanogenic, sulfate-reducing bacteria), can compete with Dehalococcoides strains for electron donors in the subsurface environment. Hence, the dynamic and long-term interactions between different microorganisms and their effects on TCE removal should be investigated thoroughly.
Currently, the genetic mechanisms associated with the anaerobic and aerobic degradation of TCE remain unclear. The integration of genomics, metagenomics, proteomics, and systems biology is a powerful tool to disclose novel bacterial functional genes of TCE degradation bacteria and provide useful information for further environmental bioremediation. Furthermore, advanced molecular biology techniques (such as compound-specific isotope analysis, fluorescence in situ hybridization, qPCR, and stable isotope probing) need to be developed and applied to evaluate the in situ biodegradation processes of TCE.
Long-term biodegradation of TCE can lead to accumulation of toxic products. Therefore, a combination of physicochemical and microbial degradation is necessary to promote the complete dehalogenation of TCE. For example, material- or nanomaterial-mediated enhanced biodegradation is a promising technique. Furthermore, the underlying mechanisms of the combined and enhanced biodegradation should be investigated comprehensively. Besides, more green and sustainable technologies need to be developed for TCE in situ remediation. Since natural attenuation is a low-cost and highly sustainable technology that integrates a series of naturally occurring biological, physical, and chemical processes (biodegradation, adsorption, volatilization, etc.), it should be widely adopted in field-scale studies.
A few anaerobic and aerobic bacterial isolates and mixed cultures have been reported to degrade TCE under laboratory conditions by batch, microcosmic experiments, and bioreactors. Further in situ studies (especially studies combining biostimulation and bioaugmentation) are needed to evaluate the biodegradation efficiency of functional microorganisms in field applications. It is worth noting that most of the field application was done in the USA, mainly around the 2000s. Therefore, current research should pay more attention to field studies and developing countries.
7. Conclusion
Biodegradation serves as a great promise in dealing with TCE pollution. Anaerobic reductive dechlorination, anaerobic cometabolic reductive dichlorination, aerobic co-metabolism, and aerobic direct metabolism are the four major biodegradation processes leading to TCE biodegradation in bacterial isolates and mixed cultures. Enzymes play crucial roles in TCE biodegradation, such as RDase for anaerobic reductive dechlorination, and monooxygenase, dioxygenase, and hydroxylase for aerobic co-metabolism. The combination of anaerobic and aerobic biodegradation is a safe and effective method to complete TCE biodegradation. The combination of microorganisms with ZVI, modified ZVI, or biochar is an effective material-mediated TCE biodegradation technology. In addition, the current research on the biodegradation of TCE in field application mainly focuses on biostimulation, bioaugmentation, and the combination of biostimulation and bioaugmentation. Finally, the challenges and prospects of TCE biodegradation are proposed based on current research. We hope this review can provide specific guidance and recommendations for future laboratory and field studies of CAHs biodegradation.
Author contributions
ZW: conceived and designed the manuscript, drafted the manuscript, reviewed and polished the article, and funding acquisition. QM: methodology, software, and reviewed and polished the article. HN, HS, and RL: methodology and reviewed and polished the article. HL: methodology, reviewed and polished the article, and funding acquisition. GR: reviewed and polished the article and funding acquisition. FZ, CP, and BL: reviewed and polished the article. XM: designed the manuscript, reviewed and polished the article, and funding acquisition. All authors contributed to the article and approved the submitted version.
Funding
The authors gratefully acknowledge financial support for this work from the Ministry of Science and Technology of the People’s Republic of China (2020YFC1808603), the National Natural Science Foundation of China (22106037, 42177218, 21876042, and 22106036), and the S&T Program of Hebei (21374204D and 22374206D).
Conflict of interest
The authors declare that the research was conducted in the absence of any commercial or financial relationships that could be construed as a potential conflict of interest.
Publisher’s note
All claims expressed in this article are solely those of the authors and do not necessarily represent those of their affiliated organizations, or those of the publisher, the editors and the reviewers. Any product that may be evaluated in this article, or claim that may be made by its manufacturer, is not guaranteed or endorsed by the publisher.
References
Abu Hamed, T., Bayraktar, E., Mehmetoglu, T., and Mehmetoglu, U. (2013). Cometabolic degradation of trichloroethylene by single- and two-phase systems. Soil Sediment Contam. 22, 85–94. doi: 10.1080/15320383.2012.714422
Aktaş, Ö., Schmidt, K. R., Mungenast, S., Stoll, C., and Tiehm, A. (2012). Effect of chloroethene concentrations and granular activated carbon on reductive dechlorination rates and growth of Dehalococcoides spp. Bioresour. Technol. 103, 286–292. doi: 10.1016/j.biortech.2011.09.119
Anderson, J. E., and McCarthy, P. L. (1997). Transformation yields of chlorinated ethenes by a methanotrophic mixed culture expressing particulate methane monooxygenase. Appl. Environ. Microbiol. 63, 687–693. doi: 10.1128/aem.63.2.687-693.1997
Applegate, B., Kelly, C., Lackey, L., McPherson, J., Kehrmeyer, S., Menn, F. M., et al. (1997). Pseudomonas putida B2: a tod-lux bioluminescent reporter for toluene and trichloroethylene co-metabolism. J. Ind. Microbiol. Biotechnol. 18, 4–9. doi: 10.1038/sj.jim.2900334
Arcangeli, J. P., and Arvin, E. (1997). Modeling of the cometabolic biodegradation of trichloroethylene by toluene oxidizing bacteria in a biofilm system. Environ. Sci. Technol. 31, 3044–3052. doi: 10.1021/es9609112
Arciero, D., Vannelli, T., Logan, M., and Hooper, A. B. (1989). Degradation of trichloroethylene by the ammonia-oxidizing bacterium Nitrosomonas europaea. Biochem. Biophys. Res. Commun. 159, 640–643. doi: 10.1016/0006-291X(89)90042-9
Arp, D. J. (1995). Understanding the diversity of trichloroethene co-oxidations. Curr. Opin. Biotechnol. 6, 352–358. doi: 10.1016/0958-1669(95)80059-X
Arp, D. J., Yeager, C. M., and Hyman, M. R. (2001). Molecular and cellular fundamentals of aerobic cometabolism of trichloroethylene. Biodegradation 12, 81–103. doi: 10.1023/a:1012089908518
Asai, M., Yoshida, N., Kusakabe, T., Ismaeil, M., Nishiuchi, T., and Katayama, A. (2022). Dehalococcoides mccartyi NIT01, a novel isolate, dechlorinates high concentrations of chloroethenes by expressing at least six different reductive dehalogenases. Environ. Res. 207:112150. doi: 10.1016/j.envres.2021.112150
Baskaran, D., and Rajamanickam, R. (2019). Aerobic biodegradation of trichloroethylene by consortium microorganism from Turkey litter compost. J. Environ. Chem. Eng. 7:103260. doi: 10.1016/j.jece.2019.103260
Berrelleza-Valdez, F., Parades-Aguilar, J., Pena-Limon, C. E., Teresa Certucha-Barragan, M., Gamez-Meza, N., Serrano-Palacios, D., et al. (2019). A novel process of the isolation of nitrifying bacteria and their development in two different natural lab-scale packed-bed bioreactors for trichloroethylene bioremediation. J. Environ. Manag. 241, 211–218. doi: 10.1016/j.jenvman.2019.04.037
Bhatt, P., Kumar, M. S., Mudliar, S., and Chakrabarti, T. (2007). Biodegradation of chlorinated compounds - a review. Crit. Rev. Environ. Sci. Technol. 37, 165–198. doi: 10.1080/10643380600776130
Bouwer, E. J., and McCarty, P. L. (1983). Transformations of 1- and 2-carbon halogenated aliphatic organic compounds under methanogenic conditions. Appl. Environ. Microbiol. 45, 1286–1294.
Brar, S. K., and Gupta, S. K. (2000). Biodegradation of trichloroethylene in a rotating biological contactor. Water Res. 34, 4207–4214. doi: 10.1016/S0043-1354(00)00183-4
Brockman, F. J., Payne, W., Workman, D. J., Soong, A., Manley, S., and Hazen, T. C. (1995). Effect of gaseous nitrogen and phosphorus injection on in situ bioremediation of a trichloroethylene-contaminated site. J. Hazard. Mater. 41, 287–298. doi: 10.1016/0304-3894(94)00119-2
Bruton, T. A., Pycke, B. F. G., and Halden, R. U. (2015). Effect of nanoscale zero-valent iron treatment on biological reductive dechlorination: a review of current understanding and research needs. Crit. Rev. Environ. Sci. Technol. 45, 1148–1175. doi: 10.1080/10643389.2014.924185
Chang, H. L., and Alvarez-Cohen, L. (1995). Transformation capacities of chlorinated organics by mixed cultures enriched on methane, propane, toluene, or phenol. Biotechnol. Bioeng. 45, 440–449. doi: 10.1002/bit.260450509
Chang, Y. C., Reddy, M. V., and Choi, D. (2021). Cometabolic degradation of toxic trichloroethene or cis-1,2-dichloroethene with phenol and production of poly-beta-hydroxybutyrate (PHB). Green Chem. 23, 2729–2737. doi: 10.1039/D1GC00265A
Chee, G. J. (2011). Biodegradation analyses of trichloroethylene (TCE) by bacteria and its use for biosensing of TCE. Talanta 85, 1778–1782. doi: 10.1016/j.talanta.2011.07.002
Chen, Y. M., Lin, T. F., Huang, C., Lin, J. C., and Hsieh, F. M. (2007). Degradation of phenol and TCE using suspended and chitosan-bead immobilized Pseudomonas putida. J. Hazard. Mater. 148, 660–670. doi: 10.1016/j.jhazmat.2007.03.030
Chen, G., Murdoch, F. K., Xie, Y., Murdoch, R. W., Cui, Y., Yang, Y., et al. (2022). Dehalogenation of chlorinated ethenes to ethene by a novel isolate, “Candidatus Dehalogenimonas etheniformans”. Appl. Environ. Microbiol. 88:e0044322. doi: 10.1128/aem.00443-22
Chen, W. Y., and Wu, J. H. (2022). Microbiome composition resulting from different substrates influences trichloroethene dechlorination performance. J. Environ. Manag. 303:114145. doi: 10.1016/j.jenvman.2021.114145
Chen, K. F., Yeh, T. Y., Kao, C. M., Sung, W. P., and Lin, C. C. (2012). Application of nanoscale zero-valent iron (nZVI) to enhance microbial reductive dechlorination of TCE: a feasibility study. Curr. Nanosci. 8, 55–59. doi: 10.2174/1573413711208010055
Cheng, D., and He, J. (2009). Isolation and characterization of “Dehalococcoides” sp strain MB, which dechlorinates tetrachloroethene to trans-1,2-Dichloroethene. Appl. Environ. Microbiol. 75, 5910–5918. doi: 10.1128/AEM.00767-09
Clark, K., Taggart, D. M., Baldwin, B. R., Ritalahti, K. M., Murdoch, R. W., Hatt, J. K., et al. (2018). Normalized quantitative PCR measurements as predictors for ethene formation at sites impacted with chlorinated ethenes. Environ. Sci. Technol. 52, 13410–13420. doi: 10.1021/acs.est.8b04373
Conrad, M. E., Brodie, E. L., Radtke, C. W., Bill, M., Delwiche, M. E., Lee, M. H., et al. (2010). Field evidence for co-metabolism of trichloroethene stimulated by addition of electron donor to groundwater. Environ. Sci. Technol. 44, 4697–4704. doi: 10.1021/es903535j
Cutright, T. J., and Meza, L. (2007). Evaluation of the aerobic biodegradation of trichloroethylene via response surface methodology. Environ. Int. 33, 338–345.
Dabrock, B., Riedel, J., Bertram, J., and Gottschalk, G. (1992). Isopropylbenzene (cumene)--a new substrate for the isolation of trichloroethene-degrading bacteria. Arch. Microbiol. 158, 9–13. doi: 10.1007/BF00249058
de Guzman, G. T. N., Hapeman, C. J., Millner, P. D., Torrents, A., Jackson, D., and Kjellerup, B. V. (2018). Presence of organohalide-respiring bacteria in and around a permeable reactive barrier at a trichloroethylene-contaminated superfund site. Environ. Pollut. 243, 766–776. doi: 10.1016/j.envpol.2018.08.095
Dey, K., and Roy, P. (2009). Degradation of trichloroethylene by bacillus sp.: isolation strategy, strain characteristics, and cell immobilization. Curr. Microbiol. 59, 256–260. doi: 10.1007/s00284-009-9427-6
Ding, C., Rogers, M. J., and He, J. (2020). Dehalococcoides mccartyi strain GEO12 has a natural tolerance to chloroform inhibition. Environ. Sci. Technol. 54, 8750–8759. doi: 10.1021/acs.est.0c00993
Dolinova, I., Strojsova, M., Cernik, M., Nemecek, J., Machackova, J., and Sevcu, A. (2017). Microbial degradation of chloroethenes: a review. Environ. Sci. Pollut. Res. 24, 13262–13283. doi: 10.1007/s11356-017-8867-y
Dong, H., Li, L., Lu, Y., Cheng, Y., Wang, Y., Ning, Q., et al. (2019). Integration of nanoscale zero-valent iron and functional anaerobic bacteria for groundwater remediation: a review. Environ. Int. 124, 265–277. doi: 10.1016/j.envint.2019.01.030
Duba, A. G., Jackson, K. J., Jovanovich, M. C., Knapp, R. B., and Taylor, R. T. (1996). TCE remediation using in situ, resting-state bioaugmentation. Environ. Sci. Technol. 30, 1982–1989. doi: 10.1021/es950730k
Dugat-Bony, E., Biderre-Petit, C., Jaziri, F., David, M. M., Denonfoux, J., Lyon, D. Y., et al. (2012). In situ TCE degradation mediated by complex dehalorespiring communities during biostimulation processes. Microb. Biotechnol. 5, 642–653. doi: 10.1111/j.1751-7915.2012.00339.x
Duhamel, M., Wehr, S. D., Yu, L., Rizvi, H., Seepersad, D., Dworatzek, S., et al. (2002). Comparison of anaerobic dechlorinating enrichment cultures maintained on tetrachloroethene, trichloroethene, cis-dichloroethene and vinyl chloride. Water Res. 36, 4193–4202. doi: 10.1016/S0043-1354(02)00151-3
Dutta, N., Thomsen, K., and Ahring, B. K. (2022). Degrading chlorinated aliphatics by reductive dechlorination of groundwater samples from the Santa Susana field laboratory. Chemosphere 298:134115. doi: 10.1016/j.chemosphere.2022.134115
Ebrahimbabaie, P., and Pichtel, J. (2021). Biotechnology and nanotechnology for remediation of chlorinated volatile organic compounds: current perspectives. Environ. Sci. Pollut. Res. 28, 7710–7741. doi: 10.1007/s11356-020-11598-y
Ensign, S. A., Hyman, M. R., and Arp, D. J. (1992). Cometabolic degradation of chlorinated alkenes by alkene monooxygenase in a propylene-grown Xanthobacter strain. Appl. Environ. Microbiol. 58, 3038–3046. doi: 10.1128/aem.58.9.3038-3046.1992
Fincker, M., and Spormann, A. M. (2017) Biochemistry of Catabolic Reductive Dehalogenation. Annu. Rev. Biochem. 86, 357–386. doi: 10.1146/annurev-biochem-061516-044829
Fitch, M. W., Weissman, D., Phelps, P., Georgiou, G., and Speitel, G. E. (1996). Trichloroethylene degradation by Methylosinus trichosporium OB3b mutants in a sequencing biofilm reactor. Water Res. 30, 2655–2664.
Fogel, M. M., Taddeo, A. R., and Fogel, S. (1986). Biodegradation of chlorinated ethenes by a methane-utilizing mixed culture. Appl. Environ. Microbiol. 51, 720–724. doi: 10.1128/aem.51.4.720-724.1986
Fox, B. G., Borneman, J. G., Wackett, L. P., and Lipscomb, J. D. (1990). Haloalkene oxidation by the soluble methane monooxygenase from Methylosinus trichosporium OB3b: mechanistic and environmental implications. Biochemistry 29, 6419–6427. doi: 10.1021/bi00479a013
Frascari, D., Fraraccio, S., Nocentini, M., and Pinelli, D. (2013). Aerobic/anaerobic/aerobic sequenced biodegradation of a mixture of chlorinated ethenes, ethanes and methanes in batch bioreactors. Bioresour. Technol. 128, 479–486. doi: 10.1016/j.biortech.2012.10.026
Freeborn, R. A., West, K. A., Bhupathiraju, V. K., Chauhan, S., and Rahm, B. G. (2005). Phylogenetic analysis of TCE-dechlorinating consortia enriched on a variety of electron donors. Environ. Sci. Technol. 39, 8358–8368. doi: 10.1021/es048003p
Fries, M. R., Hopkins, G. D., McCarty, P. L., Forney, L. J., and Tiedje, J. M. (1997). Microbial succession during a field evaluation of phenol and toluene as the primary substrates for trichloroethene cometabolism. Appl. Environ. Microbiol. 63, 1515–1522. doi: 10.1128/aem.63.4.1515-1522.1997
Futamata, H., Harayama, S., and Watanabe, K. (2001). Diversity in kinetics of trichloroethylene-degrading activities exhibited by phenol-degrading bacteria. Appl. Microbiol. Biotechnol. 55, 248–253. doi: 10.1007/s002530000500
Futamata, H., Nagano, Y., Watanabe, K., and Hiraishi, A. (2005). Unique kinetic properties of phenol-degrading Variovoyax strains responsible for efficient trichloroethylene degradation in a chemostat enrichment culture. Appl. Environ. Microbiol. 71, 904–911. doi: 10.1128/AEM.71.2.904-911.2005
Gafni, A., Siebner, H., and Bernstein, A. (2020). Potential for co-metabolic oxidation of TCE and evidence for its occurrence in a large-scale aquifer survey. Water Res. 171:115431. doi: 10.1016/j.watres.2019.115431
Gantzer, C. J., and Wackett, L. P. (1991). Reductive dechlorination catalyzed by bacterial transition-metal coenzymes. Environ. Sci. Technol. 25, 715–722. doi: 10.1021/es00016a017
Gaza, S., Schmidt, K. R., Weigold, P., Heidinger, M., and Tiehm, A. (2019). Aerobic metabolic trichloroethene biodegradation under field-relevant conditions. Water Res. 151, 343–348. doi: 10.1016/j.watres.2018.12.022
Ge, J., Huang, S., Han, I., and Jaffe, P. R. (2019). Degradation of tetra- and trichloroethylene under iron reducing conditions by Acidimicrobiaceae sp. A6. Environ. Pollut. 247, 248–255. doi: 10.1016/j.envpol.2019.01.066
Gushgari-Doyle, S., and Alvarez-Cohen, L. (2020). Effects of arsenic on Trichloroethene-Dechlorination activities of Dehalococcoides mccartyi 195. Environ. Sci. Technol. 54, 1276–1285. doi: 10.1021/acs.est.9b06527
Halsey, K. H., Sayavedra-Soto, L. A., Bottomley, P. J., and Arp, D. J. (2005). Trichloroethylene degradation by butane-oxidizing bacteria causes a spectrum of toxic effects. Appl. Microbiol. Biotechnol. 68, 794–801. doi: 10.1007/s00253-005-1944-z
Han, Y. L., Kuo, M. C. T., Tseng, I. C., and Lu, C. J. (2007). Semicontinuous microcosm study of aerobic cometabolism of trichloroethylene using toluene. J. Hazard. Mater. 148, 583–591. doi: 10.1016/j.jhazmat.2007.03.013
Hanada, S., Shigematsu, T., Shibuya, K., Eguchi, M., Hasegawa, T., Suda, F., et al. (1998). Phylogenetic analysis of trichloroethylene-degrading bacteria newly isolated from soil polluted with this contaminant. J. Ferment. Bioeng. 86, 539–544. doi: 10.1016/S0922-338X(99)80003-8
Hansen, J., Sallmen, M., Selden, A. I., Anttila, A., Pukkala, E., Andersson, K., et al. (2013). Risk of cancer among workers exposed to trichloroethylene: analysis of three nordic cohort studies. J. Nat. Can. Inst. 105, 869–877. doi: 10.1093/jnci/djt107
Harding-Marjanovic, K. C., Yi, S., Weathers, T. S., Sharp, J. O., Sedlak, D. L., and Alvarez-Cohen, L. (2016). Effects of aqueous film-forming foams (AFFFs) on trichloroethene (TCE) dechlorination by a Dehalococcoides mccartyi-containing microbial community. Environ. Sci. Technol. 50, 3352–3361. doi: 10.1021/acs.est.5b04773
Harker, A. R., and Kim, Y. (1990). Trichloroethylene degradation by two independent aromatic-degrading pathways in Alcaligenes eutrophus JMP134. Appl. Environ. Microbiol. 56, 1179–1181.
He, J., Ritalahti, K. M., Yang, K.-L., Koenigsberg, S. S., and Loffler, F. E. (2003). Detoxification of vinyl chloride to ethene coupled to growth of an anaerobic bacterium. Nature 424, 62–65. doi: 10.1038/nature01717
He, J., Sung, Y., Krajmalnik-Brown, R., Ritalahti, K. M., and Loffler, F. E. (2005). Isolation and characterization of Dehalococcoides sp strain FL2, a trichloroethene (TCE)- and 1,2-dichloroethene-respiring anaerobe. Environ. Microbiol. 7, 1442–1450. doi: 10.1111/j.1462-2920.2005.00830.x
Holliger, C., Hahn, D., Harmsen, H., Ludwig, W., Schumacher, W., Tindall, B., et al. (1998). Dehalobacter restrictus gen. Nov. and sp. nov., a strictly anaerobic bacterium that reductively dechlorinates tetra- and trichloroethene in an anaerobic respiration. Arch. Microbiol. 169, 313–321. doi: 10.1007/s002030050577
Honetschlägerová, L., Martinec, M., and Škarohlíd, R. (2019). Coupling in situ chemical oxidation with bioremediation of chloroethenes: a review. Rev. Environ. Sci. Biotechnol. 18, 699–714. doi: 10.1007/s11157-019-09512-1
Honetschlägerová, L., Škarohlíd, R., Martinec, M., Šír, M., and Luciano, V. (2018). Interactions of nanoscale zero valent iron and iron reducing bacteria in remediation of trichloroethene. Int. Biodeterior. Biodegradation 127, 241–246. doi: 10.1016/j.ibiod.2017.10.009
Hood, E. D., Quinn, D., Yoon, W. S., Gavaskar, A., and Edwards, E. A. (2008). Demonstration of enhanced bioremediation in a TCE source area at launch complex 34, Cape Canaveral air Force Station. Ground Water Monit. Remidiat. 28, 98–107. doi: 10.1111/j.1745-6592.2008.00197.x
Hopkins, G. D., and McCarty, P. L. (1995). Field evaluation of in situ aerobic cometabolism of trichloroethylene and three dichloroethylene isomers using phenol and toluene as the primary substrates. Environ. Sci. Technol. 29, 1628–1637. doi: 10.1021/es00006a029
Hu, M., Zhang, Y., Liu, Y., Wang, X., and Wong, P.-K. (2013). Effect of different nutrients on the anaerobic degradation of trichloroethene at optimal temperature. Int. Biodeterior. Biodegradation 85, 103–107. doi: 10.1016/j.ibiod.2013.06.008
Hu, M., Zhang, Y., Wang, Z., Jiang, Z., and Li, J. (2011). Influence of humic acid on the trichloroethene degradation by Dehalococcoides-containing consortium. J. Hazard. Mater. 190, 1074–1078. doi: 10.1016/j.jhazmat.2011.03.088
Huang, B., Lei, C., Wei, C., and Zeng, G. (2014). Chlorinated volatile organic compounds (Cl-VOCs) in environment - sources, potential human health impacts, and current remediation technologies. Environ. Int. 71, 118–138. doi: 10.1016/j.envint.2014.06.013
Hunt, J. R., Holden, P. A., and Firestone, M. K. (1995). Coupling transport and biodegradation of VOCs in surface and subsurface soils. Environ. Health Perspect. 103 Suppl 5, 75–78.
Ise, K., Suto, K., and Inoue, C. (2011). Microbial diversity and changes in the distribution of dehalogenase genes during dechlorination with different concentrations of cis-DCE. Environ. Sci. Technol. 45, 5339–5345. doi: 10.1021/es104199y
Islam, S., Redwan, A., Millerick, K., Filip, J., Fan, L., and Yan, W. (2021). Effect of copresence of zerovalent iron and sulfate reducing bacteria on reductive dechlorination of trichloroethylene. Environ. Sci. Technol. 55, 4851–4861. doi: 10.1021/acs.est.0c07702
Jahng, D., and Wood, T. K. (1994). Trichloroethylene and chloroform degradation by a recombinant pseudomonad expressing soluble methane monooxygenase from Methylosinus trichosporium OB3b. Appl. Environ. Microbiol. 60, 2473–2482. doi: 10.1128/aem.60.7.2473-2482.1994
Johnson, P. D., Dawson, B. V., and Goldberg, S. J. (1998). A review: trichloroethylene metabolites: potential cardiac teratogens. Environ. Health Perspect. 106, 995–999.
Jugder, B. E., Ertan, H., Lee, M., Manefield, M., and Marquis, C. P. (2015). Reductive dehalogenases come of age in biological destruction of organohalides. Trends Biotechnol. 33, 595–610. doi: 10.1016/j.tibtech.2015.07.004
Kang, J. W., Khan, Z., and Doty, S. L. (2012). Biodegradation of trichloroethylene by an endophyte of hybrid poplar. Appl. Environ. Microbiol. 78, 3504–3507. doi: 10.1128/AEM.06852-11
Kao, C. M., Liao, H. Y., Chien, C. C., Tseng, Y. K., Tang, P., Lin, C. E., et al. (2016). The change of microbial community from chlorinated solvent-contaminated groundwater after biostimulation using the metagenome analysis. J. Hazard. Mater. 302, 144–150. doi: 10.1016/j.jhazmat.2015.09.047
Kikuchi, T., Iwasaki, K., Nishihara, H., Takamura, Y., and Yagi, O. (2002). Quantitative and rapid detection of the trichloroethylene-degrading bacterium Methylocystis sp M in groundwater by real-time PCR. Appl. Microbiol. Biotechnol. 59, 731–736. doi: 10.1007/s00253-002-1087-4
Kocamemi, B. A., and Cecen, F. (2010). Biological removal of the xenobiotic trichloroethylene (TCE) through cometabolism in nitrifying systems. Bioresour. Technol. 101, 430–433. doi: 10.1016/j.biortech.2009.07.079
Kocur, C. M. D., Lomheim, L., Boparai, H. K., Chowdhury, A. I. A., Weber, K. P., Austrins, L. M., et al. (2015). Contributions of abiotic and biotic dechlorination following carboxymethyl cellulose stabilized nanoscale zero valent iron injection. Environ. Sci. Technol. 49, 8648–8656. doi: 10.1021/acs.est.5b00719
Kocur, C. M., Lomheim, L., Molenda, O., Weber, K. P., Austrins, L. M., Sleep, B. E., et al. (2016). Long-term field study of microbial community and dechlorinating activity following carboxymethyl cellulose-stabilized nanoscale zero-valent iron injection. Environ. Sci. Technol. 50, 7658–7670. doi: 10.1021/acs.est.6b01745
Koenig, J., Lee, M., and Manefield, M. (2015). Aliphatic organochlorine degradation in subsurface environments. Rev. Environ. Sci. Biotechnol. 14, 49–71. doi: 10.1007/s11157-014-9345-3
Koner, S., Chen, J. S., Hsu, B. M., Rathod, J., Huang, S. W., Chien, H. Y., et al. (2022). Depth-resolved microbial diversity and functional profiles of trichloroethylene-contaminated soils for Biolog EcoPlate-based biostimulation strategy. J. Hazard. Mater. 424:127266. doi: 10.1016/j.jhazmat.2021.127266
Kong, J. Y., Bai, Y., Su, Y., Yao, Y., and He, R. (2014). Effects of trichloroethylene on community structure and activity of methanotrophs in landfill cover soils. Soil Biol. Biochem. 78, 118–127. doi: 10.1016/j.soilbio.2014.07.018
Krajmalnik-Brown, R., Holscher, T., Thomson, I. N., Saunders, F. M., Ritalahti, K. M., and Loffler, F. E. (2004). Genetic identification of a putative vinyl chloride reductase in Dehalococcoides sp. strain BAV1. Appl. Environ. Microbiol. 70, 6347–6351. doi: 10.1128/AEM.70.10.6347-6351.2004
Kunze, C., Bommer, M., Hagen, W. R., Uksa, M., Dobbek, H., Schubert, T., et al. (2017). Cobamide-mediated enzymatic reductive dehalogenation via long-range electron transfer. Nat. Commun. 8:15858. doi: 10.1038/ncomms15858
Kuo, M. C. T., Liang, K. F., Han, Y. L., and Fan, K. C. (2004). Pilot studies for in-situ aerobic cometabolism of trichloroethylene using toluene-vapor as the primary substrate. Water Res. 38, 4125–4134. doi: 10.1016/j.watres.2004.08.009
Landa, A. S., Sipkema, E. M., Weijma, J., Beenackers, A. A. C. M., Dolfing, J., and Janssen, D. B. (1994). Cometabolic degradation of trichloroethylene by Pseudomonas cepacia G4 in a chemostat with toluene as the primary substrate. Appl. Environ. Microbiol. 60, 3368–3374. doi: 10.1128/aem.60.9.3368-3374.1994
Lee, P., Cheng, D., He, J., West, K. A., and Alvarez-Cohen, L. (2013). Isolation of two new Dehalococcoides mccartyi strains with dissimilar dechlorination functions and their characterization by comparative genomics via microarray analysis. Environ. Microbiol. 15, 2293–2305. doi: 10.1111/1462-2920.12099
Lee, P. K. H., Cheng, D., Hu, P., West, K. A., Dick, G. J., Brodie, E. L., et al. (2011). Comparative genomics of two newly isolated Dehalococcoides strains and an enrichment using a genus microarray. ISME J. 5, 1014–1024. doi: 10.1038/ismej.2010.202
Lee, M. H., Clingenpeel, S. C., Leiser, O. P., Wymore, R. A., Sorenson, K. S. Jr., and Watwood, M. E. (2008). Activity-dependent labeling of oxygenase enzymes in a trichloroethene-contaminated groundwater site. Environ. Pollut. 153, 238–246. doi: 10.1016/j.envpol.2007.07.034
Lee, C. Y., and Liu, W. D. (2006). The effect of salinity conditions on kinetics of trichloroethylene biodegradation by toluene-oxidizing cultures. J. Hazard. Mater. 137, 541–549. doi: 10.1016/j.jhazmat.2006.02.031
Lee, P. K. H., Warnecke, F., Brodie, E. L., Macbeth, T. W., Conrad, M. E., Andersen, G. L., et al. (2012). Phylogenetic microarray analysis of a microbial community performing reductive dechlorination at a TCE-contaminated site. Environ. Sci. Technol. 46, 1044–1054. doi: 10.1021/es203005k
Li, H., Chen, S., Ren, L. Y., Zhou, L. Y., Tan, X. J., Zhu, Y., et al. (2019a). Biochar mediates activation of aged nanoscale ZVI by Shewanella putrefaciens CN32 to enhance the degradation of Pentachlorophenol. Chem. Eng. J. 368, 148–156. doi: 10.1016/j.cej.2019.02.099
Li, Y., Li, B., Wang, C.-P., Fan, J.-Z., and Sun, H.-W. (2014). Aerobic degradation of trichloroethylene by co-metabolism using phenol and gasoline as growth substrates. Int. J. Mol. Sci. 15, 9134–9148. doi: 10.3390/ijms15059134
Li, J., Lu, Q., de Toledo, R. A., Lu, Y., and Shim, H. (2015). Effect of toluene concentration and hydrogen peroxide on pseudomonas plecoglossicida cometabolizing mixture of cis-DCE and TCE in soil slurry. Environ. Geochem. Health 37, 985–995. doi: 10.1007/s10653-015-9707-y
Li, Y., Wen, L. L., Zhao, H. P., and Zhu, L. (2019c). Addition of Shewanella oneidensis MR-1 to the dehalococcoides-containing culture enhances the trichloroethene dechlorination. Environ. Int. 133:105245. doi: 10.1016/j.envint.2019.105245
Li, P., Zhang, Y., Meng, Q., Liu, Y., Tuyiringire, D., Chen, Z., et al. (2019b). Effects of trichloroethylene stress on the microbiological characteristics of Mollisol. Ecotoxicol. Environ. Saf. 184:109595. doi: 10.1016/j.ecoenv.2019.109595
Li, P., Zhang, Y., Meng, Q., Liu, Y., Tuyiringire, D., Chen, Z., et al. (2020). Trichloroethylene inhibits nitrogen transformation and microbial community structure in Mollisol. Ecotoxicology 29, 801–813. doi: 10.1007/s10646-020-02230-3
Li, Y., Zhao, H. P., and Zhu, L. (2021). Iron sulfide enhanced the dechlorination of trichloroethene by Dehalococcoides mccartyi strain 195. Front. Microbiol. 12:665281. doi: 10.3389/fmicb.2021.665281
Liang, S. H., Liu, J. K., Lee, K. H., Kuo, Y. C., and Kao, C. M. (2011). Use of specific gene analysis to assess the effectiveness of surfactant-enhanced trichloroethylene cometabolism. J. Hazard. Mater. 198, 323–330. doi: 10.1016/j.jhazmat.2011.10.050
Lihl, C., Douglas, L. M., Franke, S., Perez-de-Mora, A., Meyer, A. H., Daubmeier, M., et al. (2019). Mechanistic dichotomy in bacterial trichloroethene dechlorination revealed by carbon and chlorine isotope effects. Environ. Sci. Technol. 53, 4245–4254. doi: 10.1021/acs.est.8b06643
Lin, W. H., Chien, C. C., Lu, C. W., Hou, D., Sheu, Y. T., Chen, S. C., et al. (2021). Growth inhibition of methanogens for the enhancement of TCE dechlorination. Sci. Total Environ. 787:147648. doi: 10.1016/j.scitotenv.2021.147648
Liu, J., Amemiya, T., Chang, Q., Qian, Y., and Itoh, K. (2012). Toluene dioxygenase expression correlates with trichloroethylene degradation capacity in Pseudomonas putida F1 cultures. Biodegradation 23, 683–691. doi: 10.1007/s10532-012-9544-y
Liu, Y., Chen, H., Zhao, L., Li, Z., Yi, X., Guo, T., et al. (2021). Enhanced trichloroethylene biodegradation: roles of biochar-microbial collaboration beyond adsorption. Sci. Total Environ. 792:148451. doi: 10.1016/j.scitotenv.2021.148451
Liu, N., Li, H., Li, M., Ding, L., Weng, C.-H., and Dong, C.-D. (2017). Oxygen exposure effects on the dechlorinating activities of a trichloroethene-dechlorination microbial consortium. Bioresour. Technol. 240, 98–105. doi: 10.1016/j.biortech.2017.02.112
Liu, P., Wu, Y. J., Whang, L. M., Lin, T. F., and Cho, K. C. (2020). Molecular tools with statistical analysis on trichloroethylene remediation effectiveness. Int. Biodeterior. Biodegradation 154:105050. doi: 10.1016/j.ibiod.2020.105050
Lo, K. H., Lu, C. W., Lin, W. H., Chien, C. C., Chen, S. C., and Kao, C. M. (2020). Enhanced reductive dechlorination of trichloroethene with immobilized Clostridium butyricum in silica gel. Chemosphere 238:124596. doi: 10.1016/j.chemosphere.2019.124596
Loeffler, F. E., Yan, J., Ritalahti, K. M., Adrian, L., Edwards, E. A., Konstantinidis, K. T., et al. (2013). Dehalococcoides mccartyi gen. nov., sp nov., obligately organohalide-respiring anaerobic bacteria relevant to halogen cycling and bioremediation, belong to a novel bacterial class, Dehalococcoidia classis nov., order Dehalococcoidales ord. nov and family Dehalococcoidaceae fam. nov., within the phylum Chloroflexi. Int. J. Syst. Evol. Microbiol. 63, 625–635. doi: 10.1099/ijs.0.034926-0
Löffler, F. E., and Edwards, E. A. (2006). Harnessing microbial activities for environmental cleanup. Curr. Opin. Biotechnol. 17, 274–284. doi: 10.1016/j.copbio.2006.05.001
Löffler, F. E., Yan, J., Ritalahti, K. M., Adrian, L., Edwards, E. A., Konstantinidis, K. T., et al. (2013). Dehalococcoides mccartyi gen. nov., sp nov., obligately organohalide-respiring anaerobic bacteria relevant to halogen cycling and bioremediation, belong to a novel bacterial class, Dehalococcoidia classis nov., order Dehalococcoidales ord. nov and family Dehalococcoidaceae fam. nov., within the phylum Chloroflexi. Int. J. Syst. Evol. Microbiol. 63, 625–635. doi: 10.1099/ijs.0.034926-0
Lontoh, S., Zahn, J. A., DiSpirito, A. A., and Semrau, J. D. (2000). Identification of intermediates of in vivo trichloroethylene oxidation by the membrane-associated methane monooxygenase. FEMS Microbiol. Lett. 186, 109–113. doi: 10.1111/j.1574-6968.2000.tb09090.x
Lowe, M., Madsen, E. L., Schindler, K., Smith, C., Emrich, S., Robb, F., et al. (2002). Geochemistry and microbial diversity of a trichloroethene-contaminated superfund site undergoing intrinsic in situ reductive dechlorination. FEMS Microbiol. Ecol. 40, 123–134. doi: 10.1111/j.1574-6941.2002.tb00944.x
Lyew, D., and Guiot, S. (2003). Effects of aeration and organic loading rates on degradation of trichloroethylene in a methanogenic-methanotrophic coupled reactor. Appl. Microbiol. Biotechnol. 61, 206–213. doi: 10.1007/s00253-003-1224-8
Lyu, H., Tang, J., Shen, B., and Siddique, T. (2018). Development of a novel chem-bio hybrid process using biochar supported nanoscale iron sulfide composite and Corynebacterium variabile HRJ4 for enhanced trichloroethylene dechlorination. Water Res. 147, 132–141. doi: 10.1016/j.watres.2018.09.038
Magnuson, J. K., Romine, M. F., Burris, D. R., and Kingsley, M. T. (2000). Trichloroethene reductive dehalogenase from Dehalococcoides ethenogenes: sequence of tceA and substrate range characterization. Appl. Environ. Microbiol. 66, 5141–5147. doi: 10.1128/AEM.66.12.5141-5147.2000
Malachowsky, K. J., Phelps, T. J., Teboli, A. B., Minnikin, D. E., and White, D. C. (1994). Aerobic mineralization of trichloroethylene, vinyl chloride, and aromatic compounds by Rhodococcus species. Appl. Environ. Microbiol. 60, 542–548. doi: 10.1128/aem.60.2.542-548.1994
Mao, X., Oremland, R. S., Liu, T., Gushgari, S., Landers, A. A., Baesman, S. M., et al. (2017a). Acetylene fuels TCE reductive dechlorination by defined Dehalococcoides/Pelobacter consortia. Environ. Sci. Technol. 51, 2366–2372. doi: 10.1021/acs.est.6b05770
Mao, X., Polasko, A., and Alvarez-Cohen, L. (2017b). Effects of sulfate reduction on trichloroethene dechlorination by dehalococcoides-containing microbial communities. Appl. Environ. Microbiol. 83:e03384–16. doi: 10.1128/AEM.03384-16
Mao, X., Stenuit, B., Polasko, A., and Alvarez-Cohen, L. (2015). Efficient metabolic exchange and electron transfer within a syntrophic trichloroethene-degrading coculture of Dehalococcoides mccartyi 195 and Syntrophomonas wolfei. Appl. Environ. Microbiol. 81:2015. doi: 10.1128/AEM.03464-14
Mao, X., Stenuit, B., Tremblay, J., Yu, K., Tringe, S. G., and Alvarez-Cohen, L. (2019). Structural dynamics and transcriptomic analysis of Dehalococcoides mccartyi within a TCE-dechlorinating community in a completely mixed flow reactor. Water Res. 158, 146–156. doi: 10.1016/j.watres.2019.04.038
Marco-Urrea, E., Nijenhuis, I., and Adrian, L. (2011). Transformation and carbon isotope fractionation of tetra- and trichloroethene to trans-dichloroethene by Dehalococcoides sp. strain CBDB1. Environ. Sci. Technol. 45, 1555–1562. doi: 10.1021/es1023459
Mars, A. E., Houwing, J., Dolfing, J., and Janssen, D. B. (1996). Degradation of toluene and trichloroethylene by Burkholderia cepacia G4 in growth-limited fed-batch culture. Appl. Environ. Microbiol. 62, 886–891. doi: 10.1128/aem.62.3.886-891.1996
Maymó-Gatell, X., Anguish, T., and Zinder, S. H. (1999). Reductive dechlorination of chlorinated ethenes and 1,2-dichloroethane by “Dehalococcoides ethenogenes” 195. Appl. Environ. Microbiol. 65, 3108–3113. doi: 10.1128/AEM.65.7.3108-3113.1999
Maymo-Gatell, X., Chien, Y., Gossett, J. M., and Zinder, S. H. (1997). Isolation of a bacterium that reductively dechlorinates tetrachloroethene to ethene. Science 276, 1568–1571. doi: 10.1126/science.276.5318.1568
Maymó-Gatell, X., Nijenhuis, I., and Zinder, S. H. (2001). Reductive dechlorination of cis-1,2-dichloroethene and vinyl chloride by “Dehalococcoides ethenogenes”. Environ. Sci. Technol. 35, 516–521. doi: 10.1021/es001285i
McDonald, I. R., Uchiyama, H., Kambe, S., Yagi, O., and Murrell, J. C. (1997). The soluble methane monooxygenase gene cluster of the trichloroethylene-degrading methanotroph Methylocystis sp. strain M. Appl. Environ. Microbiol. 63, 1898–1904. doi: 10.1128/aem.63.5.1898-1904.1997
Meza, L., Cutright, T. J., El-Zahab, B., and Wang, P. (2003). Aerobic biodegradation of trichloroethylene using a consortium of five bacterial strains. Biotechnol. Lett. 25, 1925–1932. doi: 10.1023/B:BILE.0000003988.70824.ef
Mukherjee, P., and Roy, P. (2012). Identification and characterisation of a bacterial isolate capable of growth on trichloroethylene as the sole carbon source. Adv. Microbiol. 02, 284–294. doi: 10.4236/aim.2012.23034
Nelson, M. J., Montgomery, S. O., and Pritchard, P. H. (1988). Trichloroethylene metabolism by microorganisms that degrade aromatic compounds. Appl. Environ. Microbiol. 54, 604–606. doi: 10.1128/aem.54.2.604-606.1988
Nemir, A., David, M. M., Perrussel, R., Sapkota, A., Simonet, P., Monier, J.-M., et al. (2010). Comparative phylogenetic microarray analysis of microbial communities in TCE-contaminated soils. Chemosphere 80, 600–607. doi: 10.1016/j.chemosphere.2010.03.036
Oldenhuis, L. R., Vink, B. D., Janssen,, and Witholt, (1989). Degradation of chlorinated aliphatic hydrocarbons by Methylosinus trichosporium OB3b expressing soluble methane monooxygenase. Appl. Environ. Microbiol. 55, 2819–2826. doi: 10.1128/aem.55.11.2819-2826.1989
Palumbo, A. V., Scarborough, S. P., Pfiffner, S. M., and Phelps, T. J. (1995). Influence of nitrogen and phosphorus on the in situ bioremediation of trichloroethylene. Appl. Biochem. Biotechnol. 51-52, 635–647. doi: 10.1007/BF02933465
Pant, P., and Pant, S. (2010). A review: advances in microbial remediation of trichloroethylene (TCE). J. Environ. Sci. 22, 116–126. doi: 10.1016/S1001-0742(09)60082-6
Perez-de-Mora, A., Zila, A., McMaster, M. L., and Edwards, E. A. (2014). Bioremediation of chlorinated ethenes in fractured bedrock and associated changes in dechlorinating and nondechlorinating microbial populations. Environ. Sci. Technol. 48, 5770–5779. doi: 10.1021/es404122y
Pfiffner, S. M., Palumbo, A. V., Phelps, T. J., and Hazen, T. C. (1997). Effects of nutrient dosing on subsurface methanotrophic populations and trichloroethylene degradation. J. Ind. Microbiol. Biotechnol. 18, 204–212. doi: 10.1038/sj.jim.2900350
Pflugmacher, U., Averhoff, B., and Gottschalk, G. (1996). Cloning, sequencing, and expression of isopropylbenzene degradation genes from Pseudomonas sp. strain JR1: identification of isopropylbenzene dioxygenase that mediates trichloroethene oxidation. Appl. Environ. Microbiol. 62, 3967–3977. doi: 10.1128/aem.62.11.3967-3977.1996
Polasko, A. L., Zulli, A., Gedalanga, P. B., Pornwongthong, P., and Mahendra, S. (2019). A mixed microbial community for the biodegradation of chlorinated ethenes and 1,4-dioxane. Environ. Sci. Technol. Lett. 6, 49–54. doi: 10.1021/acs.estlett.8b00591
Pon, G., Hyman, M. R., and Semprini, L. (2003). Acetylene inhibition of trichloroethene and vinyl chloride reductive dechlorination. Environ. Sci. Technol. 37, 3181–3188. doi: 10.1021/es026352i
Popat, S. C., and Deshusses, M. A. (2011). Kinetics and inhibition of reductive dechlorination of trichloroethene, cis-1,2-dichloroethene and vinyl chloride in a continuously fed anaerobic biofilm reactor. Environ. Sci. Technol. 45, 1569–1578. doi: 10.1021/es102858t
Popat, S. C., Zhao, K., and Deshusses, M. A. (2012). Bioaugmentation of an anaerobic biotrickling filter for enhanced conversion of trichloroethene to ethene. Chem. Eng. J. 183, 98–103. doi: 10.1016/j.cej.2011.12.026
Qiu, L., Lok, K. S., Lu, Q., Zhong, H., Guo, X., and Shim, H. (2022). Zinc and copper supplements enhance trichloroethylene removal by Pseudomonas plecoglossicida in water. Environ. Technol., 1–12. doi: 10.1080/09593330.2022.2069518
Rao, T., Ma, X., Yang, Q., Cheng, S., Ren, G., Wu, Z., et al. (2022). Upgrading the peroxi-coagulation treatment of complex water matrices using a magnetically assembled mZVI/DSA anode: insights into the importance of ClO radical. Chemosphere 303:134948. doi: 10.1016/j.chemosphere.2022.134948
Rasche, M. E., Hyman, M. R., and Arp, D. J. (1991). Factors limiting aliphatic chlorocarbon degradation by Nitrosomonas europaea: Cometabolic inactivation of ammonia monooxygenase and substrate specificity. Appl. Environ. Microbiol. 57, 2986–2994. doi: 10.1128/aem.57.10.2986-2994.1991
Reij, M. W., Kieboom, J., De Bont, J. A. M., and Hartmans, S. (1995). Continuous degradation of trichloroethylene by Xanthobacter sp. strain Py2 during growth on propene. Appl. Environ. Microbiol. 61, 2936–2942. doi: 10.1128/aem.61.8.2936-2942.1995
Robles, A., Yellowman, T. L., Joshi, S., Rangan, S. M., and Delgado, A. G. (2021). Microbial chain elongation and subsequent fermentation of elongated carboxylates as H2-producing processes for sustained reductive dechlorination of chlorinated ethenes. Environ. Sci. Technol. 55, 10398–10410. doi: 10.1021/acs.est.1c01319
Rossi, M. M., Alfano, S., Amanat, N., Andreini, F., Lorini, L., Martinelli, A., et al. (2022a). A polyhydroxybutyrate (PHB)-biochar reactor for the adsorption and biodegradation of trichloroethylene: design and startup phase. Bioengineering 9:192. doi: 10.3390/bioengineering9050192
Rossi, M. M., Matturro, B., Amanat, N., Rossetti, S., and Petrangeli Papini, M. (2022b). Coupled adsorption and biodegradation of trichloroethylene on biochar from pine wood wastes: a combined approach for a sustainable bioremediation strategy. Microorganisms 10:101. doi: 10.3390/microorganisms10010101
Rupakula, A., Lu, Y., Kruse, T., Boeren, S., Holliger, C., Smidt, H., et al. (2015). Functional genomics of corrinoid starvation in the organohalide-respiring bacterium Dehalobacter restrictus strain PER-K23. Front. Microbiol. 5:751. doi: 10.3389/fmicb.2014.00751
Ryoo, D., Shim, H., Arenghi, F. L. G., Barbieri, P., and Wood, T. K. (2001). Tetrachloroethylene, trichloroethylene, and chlorinated phenols induce toluene-o-xylene monooxygenase activity in Pseudomonas stutzeri OX1. Appl. Microbiol. Biotechnol. 56, 545–549. doi: 10.1007/s002530100675
Saeki, H., Akira, M., Furuhashi, K., Averhoff, B., and Gottschalk, G. (1999). Degradation of trichloroethene by a linear-plasmid-encoded alkene monooxygenase in Rhodococcus corallinus (Nocardia corallina) B-276. Microbiology 145, 1721–1730. doi: 10.1099/13500872-145-7-1721
Semprini, L. (1997). Strategies for the aerobic co-metabolism of chlorinated solvents. Curr. Opin. Biotechnol. 8, 296–308. doi: 10.1016/S0958-1669(97)80007-9
Semrau, J. D., DiSpirito, A. A., and Yoon, S. (2010). Methanotrophs and copper. FEMS Microbiol. Rev. 34, 496–531. doi: 10.1111/j.1574-6976.2010.00212.x
Shanbhogue, S. S., Bezbaruah, A., Simsek, S., and Khan, E. (2017). Trichloroethene removal by separately encapsulated and co-encapsulated bacterial degraders and nanoscale zero-valent iron. Int. Biodeterior. Biodegradation 125, 269–276. doi: 10.1016/j.ibiod.2017.08.005
Shao, Y., Hatzinger, P. B., Streger, S. H., Rezes, R. T., and Chu, K.-H. (2019). Evaluation of methanotrophic bacterial communities capable of biodegrading trichloroethene (TCE) in acidic aquifers. Biodegradation 30, 173–190. doi: 10.1007/s10532-019-09875-w
Sheu, Y. T., Chen, S. C., Chien, C. C., Chen, C. C., and Kao, C. M. (2015). Application of a long-lasting colloidal substrate with pH and hydrogen sulfide control capabilities to remediate TCE-contaminated groundwater. J. Hazard. Mater. 284, 222–232. doi: 10.1016/j.jhazmat.2014.11.023
Shields, M. S., Montgomery, S. O., Cuskey, S. M., Chapman, P. J., and Pritchard, P. H. (1991). Mutants of pseudomonas cepacia G4 defective in catabolism of aromatic compounds and trichloroethylene. Appl. Environ. Microbiol. 57, 1935–1941. doi: 10.1128/aem.57.7.1935-1941.1991
Shimomura, T., Suda, F., Uchiyama, H., and Yagi, O. (1997). Biodegradation of trichloroethylene by Methylocystis sp. strain M immobilized in gel beads in a fluidized-bed bioreactor. Water Res. 31, 2383–2386. doi: 10.1016/S0043-1354(97)00062-6
Shin, H. Y., Singhal, N., and Park, J. W. (2007). Regeneration of iron for trichloroethylene reduction by Shewanella alga BrY. Chemosphere 68, 1129–1134. doi: 10.1016/j.chemosphere.2007.01.059
Shukla, A. K., Upadhyay, S. N., and Dubey, S. K. (2014). Current trends in trichloroethylene biodegradation: a review. Crit. Rev. Biotechnol. 34, 101–114. doi: 10.3109/07388551.2012.727080
Shukla, A. K., Vishwakarma, P., Upadhyay, S. N., Tripathi, A. K., Prasana, H. C., and Dubey, S. K. (2009). Biodegradation of trichloroethylene (TCE) by methanotrophic community. Bioresour. Technol. 100, 2469–2474. doi: 10.1016/j.biortech.2008.12.022
Siggins, A., Thorn, C., Healy, M. G., and Abram, F. (2021). Simultaneous adsorption and biodegradation of trichloroethylene occurs in a biochar packed column treating contaminated landfill leachate. J. Hazard. Mater. 403:123676. doi: 10.1016/j.jhazmat.2020.123676
Smith, K. S., Costello, A. M., and Lidstrom, M. E. (1997). Methane and trichloroethylene oxidation by an estuarine methanotroph, Methylobacter sp. strain BB5.1. Appl. Environ. Microbiol. 63, 4617–4620. doi: 10.1128/aem.63.11.4617-4620.1997
Steffan, R. J., Sperry, K. L., Walsh, M. T., Vainberg, S., and Condee, C. W. (1999). Field-scale evaluation of in situ bioaugmentation for remediation of chlorinated solvents in groundwater. Environ. Sci. Technol. 33, 2771–2781. doi: 10.1021/es9812204
Sullivan, J. P., Dickinson, D., and Chase, H. A. (1998). Methanotrophs, Methylosinus trichosporium OB3b, sMMO, and their application to bioremediation. Crit. Rev. Microbiol. 24, 335–373. doi: 10.1080/10408419891294217
Sun, A. K., Hong, J., and Wood, T. K. (1998). Modeling trichloroethylene degradation by a recombinant pseudomonad expressing toluene ortho-monooxygenase in a fixed-film bioreactor. Biotechnol. Bioeng. 59, 40–51. doi: 10.1002/(SICI)1097-0290(19980705)59:1<40::AID-BIT6>3.0.CO;2-T
Sun, A. K., and Wood, T. K. (1996). Trichloroethylene degradation and mineralization by Pseudomonads and Methylosinus trichosporium OB3b. Appl. Microbiol. Biotechnol. 45, 248–256. doi: 10.1007/s002530050679
Sung, Y., Ritalahti, K. M., Apkarian, R. P., and Loffler, F. E. (2006). Quantitative PCR confirms purity of strain GT, a novel trichloroethene-to-ethene-respiring Dehalococcoides isolate. Appl. Environ. Microbiol. 72, 1980–1987. doi: 10.1128/AEM.72.3.1980-1987.2006
Suttinun, O., Luepromchai, E., and Mueller, R. (2013). Cometabolism of trichloroethylene: concepts, limitations and available strategies for sustained biodegradation. Rev. Environ. Sci. Biotechnol. 12, 99–114. doi: 10.1007/s11157-012-9291-x
Suttinun, O., Mueller, R., and Luepromchai, E. (2010). Cometabolic degradation of trichloroethene by Rhodococcus sp. strain L4 immobilized on plant materials rich in essential oils. Appl. Environ. Microbiol. 76, 4684–4690. doi: 10.1128/AEM.03036-09
Tachachartvanich, P., Sangsuwan, R., Ruiz, H. S., Sanchez, S. S., Durkin, K. A., Zhang, L., et al. (2018). Assessment of the endocrine-disrupting effects of trichloroethylene and its metabolites using in vitro and in silico approaches. Environ. Sci. Technol. 52, 1542–1550. doi: 10.1021/acs.est.7b04832
Tang, S., Chan, W. W., Fletcher, K. E., Seifert, J., Liang, X., Loffler, F. E., et al. (2013). Functional characterization of reductive dehalogenases by using blue native polyacrylamide gel electrophoresis. Appl. Environ. Microbiol. 79, 974–981. doi: 10.1128/AEM.01873-12
Tartakovsky, B., Manuel, M. F., and Guiot, S. R. (2003). Trichloroethylene degradation in a coupled anaerobic/aerobic reactor oxygenated using hydrogen peroxide. Environ. Sci. Technol. 37, 5823–5828. doi: 10.1021/es030340v
Tian, T., and Yu, H. Q. (2020). Iron-assisted biological wastewater treatment: synergistic effect between iron and microbes. Biotechnol. Adv. 44:107610. doi: 10.1016/j.biotechadv.2020.107610
Tiehm, A., and Schmidt, K. R. (2011). Sequential anaerobic/aerobic biodegradation of chloroethenes - aspects of field application. Curr. Opin. Biotechnol. 22, 415–421. doi: 10.1016/j.copbio.2011.02.003
Tomita, R., Yoshida, N., and Meng, L. (2022). Formate: a promising electron donor to enhance trichloroethene-to-ethene dechlorination in Dehalococcoides-augmented groundwater ecosystems with minimal bacterial growth. Chemosphere 307:136080. doi: 10.1016/j.chemosphere.2022.136080
Tresse, O., Mounien, F., Levesque, M. J., and Guiot, S. (2005). Comparison of the microbial population dynamics and phylogenetic characterization of a CANOXIS reactor and a UASB reactor degrading trichloroethene. J. Appl. Microbiol. 98, 440–449. doi: 10.1111/j.1365-2672.2004.02475.x
Tsai, T. T., Liu, J. K., Chang, Y. M., Chen, K. F., and Kao, C. M. (2014). Application of polycolloid-releasing substrate to remediate trichloroethylene-contaminated groundwater: a pilot-scale study. J. Hazard. Mater. 268, 92–101. doi: 10.1016/j.jhazmat.2014.01.004
Tschantz, M. F., Bowman, J. P., Donaldson, T. L., Bienkowski, P. R., Strong-Gunderson, J. M., Palumbo, A. V., et al. (1995). Methanotrophic TCE biodegradation in a multi-stage bioreactor. Environ. Sci. Technol. 29, 2073–2082. doi: 10.1021/es00008a029
Tsien, H. C., Brusseau, G. A., Hanson, R. S., and Waclett, L. P. (1989). Biodegradation of trichloroethylene by Methylosinus trichosporium OB3b. Appl. Environ. Microbiol. 55, 3155–3161. doi: 10.1128/aem.55.12.3155-3161.1989
Underwood, J. C., Akob, D. M., Lorah, M. M., Imbrigiotta, T. E., Harvey, R. W., and Tiedeman, C. R. (2022). Microbial community response to a bioaugmentation test to degrade trichloroethylene in a fractured rock aquifer, Trenton, N.J. FEMS Microbiol. Ecol. 98, 1–16. doi: 10.1093/femsec/fiac077
Vanderberg, L. A., Burback, B. L., and Perry, J. J. (1995). Biodegradation of trichloroethylene by Mycobacterium vaccae. Can. J. Microbiol. 41, 298–301. doi: 10.1139/m95-041
Vlaanderen, J., Straif, K., Pukkala, E., Kauppinen, T., Kyyronen, P., Martinsen, J. I., et al. (2013). Occupational exposure to trichloroethylene and perchloroethylene and the risk of lymphoma, liver, and kidney cancer in four Nordic countries. Occup. Environ. Med. 70, 393–401. doi: 10.1136/oemed-2012-101188
Vogel, T. M., and McCarty, P. L. (1985). Biotransformation of tetrachloroethylene to trichloroethylene, dichloroethylene, vinyl chloride, and carbon dioxide under methanogenic conditions. Appl. Environ. Microbiol. 49, 1080–1083. doi: 10.1128/aem.49.5.1080-1083.1985
Wackett, L. P., Brusseau, G. A., Householder, S. R., and Hanson, R. S. (1989). Survey of microbial oxygenases: trichloroethylene degradation by propane-oxidizing bacteria. Appl. Environ. Microbiol. 55, 2960–2964. doi: 10.1128/aem.55.11.2960-2964.1989
Walaszek, M., Cary, L., Billon, G., Blessing, M., Bouvet-Swialkowski, A., George, M., et al. (2021). Dynamics of chlorinated aliphatic hydrocarbons in the chalk aquifer of northern France. Sci. Total Environ. 757:143742. doi: 10.1016/j.scitotenv.2020.143742
Wang, S., Chen, S., Wang, Y., Low, A., Lu, Q., and Qiu, R. (2016). Integration of organohalide-respiring bacteria and nanoscale zero-valent iron (Bio-nZVI-RD): a perfect marriage for the remediation of organohalide pollutants? Biotechnol. Adv. 34, 1384–1395. doi: 10.1016/j.biotechadv.2016.10.004
Wang, Q., Guo, S., Ali, M., Song, X., Tang, Z., Zhang, Z., et al. (2022). Thermally enhanced bioremediation: a review of the fundamentals and applications in soil and groundwater remediation. J. Hazard. Mater. 433:128749. doi: 10.1016/j.jhazmat.2022.128749
Wang, C. C., Li, C. H., and Yang, C. F. (2019). Acclimated methanotrophic consortia for aerobic co-metabolism of trichloroethene with methane. Int. Biodeterior. Biodegradation 142, 52–57. doi: 10.1016/j.ibiod.2019.05.002
Wang, S.-M., and Tseng, S.-K. (2009). Dechlorination of trichloroethylene by immobilized autotrophic hydrogen-bacteria and zero-valent iron. J. Biosci. Bioeng. 107, 287–292. doi: 10.1016/j.jbiosc.2008.11.010
Wang, X., Xin, J., Yuan, M., and Zhao, F. (2020). Electron competition and electron selectivity in abiotic, biotic, and coupled systems for dechlorinating chlorinated aliphatic hydrocarbons in groundwater: a review. Water Res. 183:116060. doi: 10.1016/j.watres.2020.116060
Wartenberg, D., Reyner, D., and Scott, C. S. (2000). Trichloroethylene and cancer: epidemiologic evidence. Environ. Health Perspect. 108, 161–176. doi: 10.1289/ehp.00108s2161
Weathers, T. S., Harding-Marjanovic, K., Higgins, C. P., Alvarez-Cohen, L., and Sharp, J. O. (2016). Perfluoroalkyl acids inhibit reductive dechlorination of trichloroethene by repressing Dehalococcoides. Environ. Sci. Technol. 50, 240–248. doi: 10.1021/acs.est.5b04854
Wen, L. L., Yang, Q., Zhang, Z. X., Yi, Y. Y., Tang, Y., and Zhao, H. P. (2016). Interaction of perchlorate and trichloroethene bioreductions in mixed anaerobic culture. Sci. Total Environ. 571, 11–17. doi: 10.1016/j.scitotenv.2016.07.122
Wen, L. L., Zhang, Y., Chen, J. X., Zhang, Z. X., Yi, Y. Y., Tang, Y., et al. (2017). The dechlorination of TCE by a perchlorate reducing consortium. Chem. Eng. J. 313, 1215–1221. doi: 10.1016/j.cej.2016.11.021
Wild, A., Hermann, R., and Leisinger, T. (1996). Isolation of an anaerobic bacterium which reductively dechlorinates tetrachloroethene and trichloroethene. Biodegradation 7, 507–511.
Wilson, J. T., and Wilson, B. H. (1985). Biotransformation of trichloroethylene in soil. Appl. Environ. Microbiol. 49, 242–243.
Winter, R. B., Yen, K. M., and Ensley, B. D. (1989). Efficient degradation of trichloroethylene by a recombinant Escherichia coli. Nat. Biotechnol. 7, 282–285. doi: 10.1038/nbt0389-282
Wohlfarth, G., and Diekert, G. (1997). Anaerobic dehalogenases. Curr. Opin. Biotechnol. 8, 290–295. doi: 10.1016/S0958-1669(97)80006-7
Wu, Z., Gao, G., and Wang, Y. (2019). Effects of soil properties, heavy metals, and PBDEs on microbial community of e-waste contaminated soil. Ecotoxicol. Environ. Saf. 180, 705–714. doi: 10.1016/j.ecoenv.2019.05.027
Wu, N. J., Zhang, W., Wei, W. X., Yang, S. C., Wang, H. J., Sun, Z. P., et al. (2020). Field study of chlorinated aliphatic hydrocarbon degradation in contaminated groundwater via micron zero-valent iron coupled with biostimulation. Chem. Eng. J. 384:123349. doi: 10.1016/j.cej.2019.123349
Xiao, Z., Jiang, W., Chen, D., and Xu, Y. (2020). Bioremediation of typical chlorinated hydrocarbons by microbial reductive dechlorination and its key players: a review. Ecotoxicol. Environ. Saf. 202:110925. doi: 10.1016/j.ecoenv.2020.110925
Xing, Z., Su, X., Zhang, X., Zhang, L., and Zhao, T. (2022). Direct aerobic oxidation (DAO) of chlorinated aliphatic hydrocarbons: a review of key DAO bacteria, biometabolic pathways and in-situ bioremediation potential. Environ. Int. 162:107165. doi: 10.1016/j.envint.2022.107165
Xiu, Z. M., Jin, Z. H., Li, T. L., Mahendra, S., Lowry, G. V., and Alvarez, P. J. J. (2010). Effects of nano-scale zero-valent iron particles on a mixed culture dechlorinating trichloroethylene. Bioresour. Technol. 101, 1141–1146. doi: 10.1016/j.biortech.2009.09.057
Yadvika, S., Sreekrishnan, T. R., Kohli, S., and Rana, V. (2004). Enhancement of biogas production from solid substrates using different techniques - a review. Bioresour. Technol. 95, 1–10. doi: 10.1016/j.biortech.2004.02.010
Yamazaki, Y., Kitamura, G., Tian, X., Suzuki, I., Kobayashi, T., Shimizu, T., et al. (2022). Temperature dependence of sequential chlorinated ethenes dechlorination and the dynamics of dechlorinating microorganisms. Chemosphere 287:131989. doi: 10.1016/j.chemosphere.2021.131989
Yan, J., Wang, J., Solis, M. I. V., Jin, H., Chourey, K., Li, X., et al. (2021). Respiratory vinyl chloride reductive dechlorination to ethene in TceA-expressing Dehalococcoides mccartyi. Environ. Sci. Technol. 55, 4831–4841. doi: 10.1021/acs.est.0c07354
Yang, Z., Wang, X.-l., Li, H., Yang, J., Zhou, L.-Y., and Liu, Y.-D. (2017). Re-activation of aged-ZVI by iron-reducing bacterium Shewanella putrefaciens for enhanced reductive dechlorination of trichloroethylene. J. Chem. Technol. Biotechnol. 92, 2642–2649, doi: 10.1002/jctb.5284.
Yang, C. E., Wu, C. Y., Liu, Y. C., Lan, E. I., and Tsai, S. L. (2019). Cometabolic degradation of toluene and TCE contaminated wastewater in a bench-scale sequencing batch reactor inoculated with immobilized Pseudomonas putida F1. J. Taiwan Inst. Chem. Eng. 104, 168–176. doi: 10.1016/j.jtice.2019.09.003
Yang, J., Zhou, L. Y., and Li, H. (2018). Synergistic effects of acclimated bacterial community and zero valent iron for removing 1,1,1-trichloroethane and 1,4-dioxane co-contaminants in groundwater. J. Chem. Technol. Biotechnol. 93, 2244–2251. doi: 10.1002/jctb.5567
Yuan, M., Xin, J., Wang, X., Zhao, F., Wang, L., and Liu, M. (2022). Coupling microscale zero-valent iron and autotrophic hydrogen-bacteria provides a sustainable remediation solution for trichloroethylene-contaminated groundwater: mechanisms, regulation, and engineering implications. Water Res. 216:118286. doi: 10.1016/j.watres.2022.118286
Zalesak, M., Ruzicka, J., Vicha, R., and Dvorackova, M. (2017). Cometabolic degradation of dichloroethenes by Comamonas testosteroni RF2. Chemosphere 186, 919–927. doi: 10.1016/j.chemosphere.2017.07.156
Zalesak, M., Ruzicka, J., Vicha, R., and Dvorackova, M. (2021). Examining aerobic degradation of chloroethenes mixture in consortium composed of Comamonas testosteroni RF2 and Mycobacterium aurum L1. Chemosphere 269:128770. doi: 10.1016/j.chemosphere.2020.128770
Zhang, S., Adrian, L., and Schueuermann, G. (2020). Dehalococcoides-mediated B-12-dependent reductive dehalogenation of aromatics does not proceed through outer-sphere electron transfer. Environ. Sci. Technol. 54, 15751–15758. doi: 10.1021/acs.est.0c04404
Zhang, S., Adrian, L., and Schüürmann, G. (2021). Outer-sphere electron transfer does not underpin B12-dependent olefinic reductive dehalogenation in anaerobes. Phys. Chem. Chem. Phys. 23, 27520–27524. doi: 10.1039/D1CP04632B
Zhang, Y., Hu, M., Li, P., Wang, X., and Meng, Q. (2015a). Analysis of trichloroethylene removal and bacterial community function based on pH-adjusted in an upflow anaerobic sludge blanket reactor. Appl. Microbiol. Biotechnol. 99, 9289–9297. doi: 10.1007/s00253-015-6800-1
Zhang, J. J., Joslyn, A. P., and Chiu, P. C. (2006). 1,1-dichloroethene as a predominant intermediate of microbial trichloroethene reduction. Environ. Sci. Technol. 40, 1830–1836. doi: 10.1021/es051829m
Zhang, Y., Wang, X., Hu, M., and Li, P. (2015b). Effect of hydraulic retention time (HRT) on the biodegradation of trichloroethylene wastewater and anaerobic bacterial community in the UASB reactor. Appl. Microbiol. Biotechnol. 99, 1977–1987. doi: 10.1007/s00253-014-6096-6
Zhao, S. Y., and He, J. Z. (2019). Reductive dechlorination of high concentrations of chloroethenes by a Dehalococcoides mccartyi strain 11G. FEMS Microbiol. Ecol. 95:fiy209. doi: 10.1093/femsec/fiy209
Zhou, Q., Li, D., Wang, T., and Hu, X. (2021). Leaching of graphene oxide nanosheets in simulated soil and their influences on microbial communities. J. Hazard. Mater. 404:124046. doi: 10.1016/j.jhazmat.2020.124046
Zhou, Q., Liu, Y., Li, T., Zhao, H., Alessi, D. S., Liu, W., et al. (2020). Cadmium adsorption to clay-microbe aggregates: implications for marine heavy metals cycling. Geochim. Cosmochim. Acta 290, 124–136. doi: 10.1016/j.gca.2020.09.002
Zhou, Q., Wang, S., Liu, J., Hu, X., Liu, Y., He, Y., et al. (2022). Geological evolution of offshore pollution and its long-term potential impacts on marine ecosystems. Geosci. Front. 13:101427. doi: 10.1016/j.gsf.2022.101427
Glossary
Keywords: anaerobic, aerobic, biodegradation, mechanism, trichloroethylene
Citation: Wu Z, Man Q, Niu H, Lyu H, Song H, Li R, Ren G, Zhu F, Peng C, Li B and Ma X (2022) Recent advances and trends of trichloroethylene biodegradation: A critical review. Front. Microbiol. 13:1053169. doi: 10.3389/fmicb.2022.1053169
Edited by:
Wenjie Ren, Institute of Soil Science (CAS), ChinaReviewed by:
Qihong Lu, Sun Yat-sen University, ChinaShangwei Zhang, Beijing Normal University, China
Copyright © 2022 Wu, Man, Niu, Lyu, Song, Li, Ren, Zhu, Peng, Li and Ma. This is an open-access article distributed under the terms of the Creative Commons Attribution License (CC BY). The use, distribution or reproduction in other forums is permitted, provided the original author(s) and the copyright owner(s) are credited and that the original publication in this journal is cited, in accordance with accepted academic practice. No use, distribution or reproduction is permitted which does not comply with these terms.
*Correspondence: Xiaodong Ma, maxd@hebut.edu.cn