- 1Department of Obstetrics and Gynecology, The Affiliated Hospital of Qingdao University, Qingdao, China
- 2Department of Cardiovascular Medicine, The Affiliated Hospital of Qingdao University, Qingdao, China
- 3Department of Medical Genetics, The Affiliated Hospital of Qingdao University, Qingdao, China
The complex pathogenesis of preeclampsia (PE), a significant contributor to maternal and neonatal mortality globally, is poorly understood despite substantial research. This review explores the involvement of exosomal microRNAs (exomiRs) in PE, focusing on their impact on the protein kinase B (AKT)/hypoxia-inducible factor 1-α (HIF1α)/vascular endothelial growth factor (VEGF) signaling pathway as well as endothelial cell proliferation and migration. Specifically, this article amalgamates existing evidence to reveal the pivotal role of exomiRs in regulating mesenchymal stem cell and trophoblast function, placental angiogenesis, the renin–angiotensin system, and nitric oxide production, which may contribute to PE etiology. This review emphasizes the limited knowledge regarding the role of exomiRs in PE while underscoring the potential of exomiRs as non-invasive biomarkers for PE diagnosis, prediction, and treatment. Further, it provides valuable insights into the mechanisms of PE, highlighting exomiRs as key players with clinical implications, warranting further exploration to enhance the current understanding and the development of novel therapeutic interventions.
1 Introduction
Preeclampsia (PE) is a syndrome characterized by hypertension and concurrent multisystemic dysfunction during pregnancy (1). It affects approximately 2–8% of pregnancies worldwide, and the incidence of PE varies among different countries and regions, with estimates ranging from at least 16% in low-income and middle-income countries to more than 25% in certain countries in Latin America (2). Globally, preeclampsia causes the loss of 76,000 pregnant women and 500,000 fetuses every year, seriously endangering the health of pregnant women and perinatal infants (3). Furthermore, previous exposure to PE is projected to increase the risk of chronic health problems for around 300 million women and children worldwide (4). This places a significant burden on families and society, making it an important global public health issue (5). According to the International Society for the Study of Hypertension in Pregnancy (ISSHP), PE can be defined as a progressive disease of pregnancy involving multiple organ systems. PE can be diagnosed after 20 weeks of gestation by new-onset hypertension (systolic blood pressure ≥140 mmHg and/or diastolic blood pressure ≥90 mmHg; average of two measurements) in a patient previously with normotension plus one other pre-eclampsia-related symptom or sign. These can include proteinuria (protein/creatinine ratio ≥30 mg/mmol in a spot urine sample or ≥300 mg/mmol in >0.3 g/day), acute kidney injury (creatinine ≥90 µmol/l), liver involvement (elevated transaminases), neurological symptoms (eclampsia, altered mental status, blindness, stroke, clonus, severe headaches, persistent visual scotomata), hematological abnormalities (thrombocytopenia, disseminated intravascular coagulation, hemolysis), cardiorespiratory complications (pulmonary edema, myocardial ischemia or infarction, oxygen saturation <90%, ≥0%, inspired oxygen for more than 1 hour, intubation other than for cesarean), or uteroplacental dysfunction (placental abruption) (4). The condition poses significant risks to maternal health, including potentially fatal outcomes such as disseminated intravascular coagulation, eclampsia, cerebral hemorrhage, pulmonary edema, acute renal damage, hepatic failure, and stroke, making PE the second leading cause of maternal death in clinics (6). A strong correlation has been reported between a history of PE and future cardiovascular or cerebrovascular disease risk, emphasizing the long-term implications of this condition in the mother (7). Moreover, PE contributes to increased fetal morbidity and mortality through iatrogenic preterm delivery, fetal growth restriction, and placental abruption (8).
Similar to ISSHP guidelines, the American College of Obstetricians and Gynecologists (ACOG) and the National Institute for Health and Care Excellence (NICE) guidelines recognize that high-risk factors include obstetric history and maternal factors (4). Moreover, NICE and ACOG have released risk assessment guidelines based on maternal characteristics and medical history. The pathogenesis of PE is multifactorial, involving two stages. The first is abnormal placentation in early pregnancy due to insufficient extravillous trophoblast (EVT) invasion and suboptimal spiral artery remodeling. The second stage comprises placental ischemia/reperfusion injury leading to maternal angiogenic imbalance, immune-mediated response, and endothelial cell dysfunction (9) (Figure 1). Despite advancements, the specific mechanisms underlying PE pathogenesis and etiology remain elusive. In particular, the accumulation of reactive oxygen species (ROS) resulting from heightened oxidative stress (OS) plays a significant role in the two stages, making it a key indicator of PE (10). Additionally, impaired spiral artery remodeling, poor placental implantation, and abnormal levels of angiogenic proteins in maternal blood are also important indicators of PE (11). The ISSHP guidelines recommend oral aspirin, low dietary calcium intake, and exercise to reduce the likelihood of PE (12). Once diagnosed, the management method involves delivering the baby and placenta at full term gestation, or, if preterm PE is detected, expectant management can be used until a more advanced gestation is reached (11).
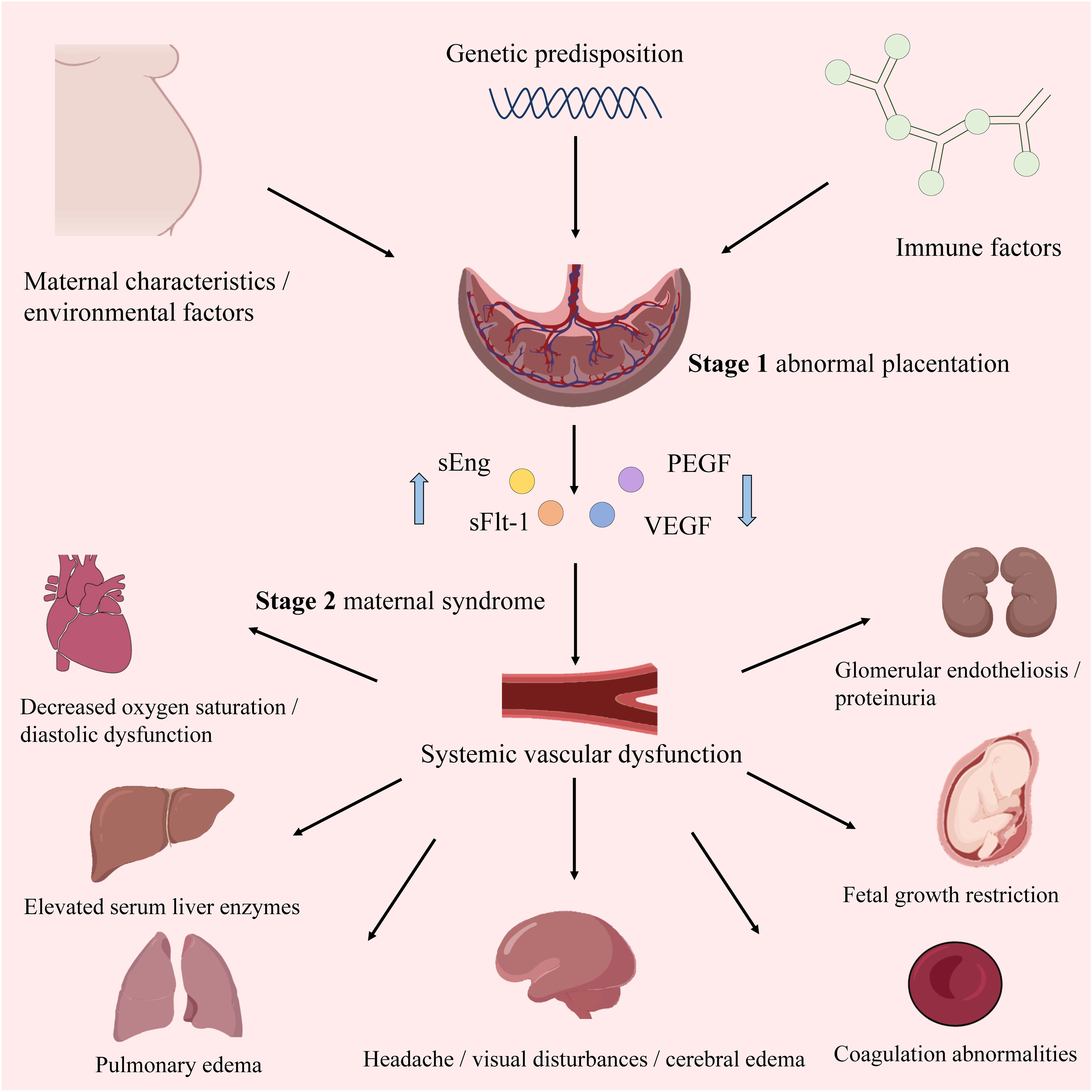
Figure 1 The overview of PE. The upward blue arrow represents “increase”, and the downward blue arrow represents “decrease”.
Current consensus suggests that PE arises from a combination of factors, including aberrant placental function, immune-system modifications, inflammatory activity, imbalanced angiogenic and anti-angiogenic factors, and metabolic abnormalities (11). Additionally, genetic variation, trace elements, changes in lipid metabolism, and OS in pregnant women have been implicated in PE pathophysiology (13). Emerging evidence points to the role of epigenetics, particularly microRNAs (miRNAs), in PE pathogenesis (14). For example, certain miRNAs (e.g., miR-182 and miR-210) are dysregulated in individuals with PE compared to normal pregnancy (15). Notably, exosomal microRNAs (exomiRs) have also been implicated in PE, including miR-153-3p and miR-517a (8). Tkachenko et al. assumed that the altered level of miR-210 could influence the expression of specific miRNAs in the development of PE, including miR-1, miR-27a, miR-29a, miR-130a, miR-152, miR-193b, and miR-519b (16). Escudero et al. proposed that the synthesis of exosomes containing miRNAs is influenced by various factors such as hypoxia and the balance between pro-oxidative and anti-oxidative mechanisms, and they may contribute to the significant changes in endothelial protein expression observed in PE, resulting in endothelial dysfunction in both maternal and maternal-fetal circulation and subsequent impairment of angiogenesis, which is the key feature of PE (1).
Owing to gaps in knowledge and limitations in early detection methods, effective preventive and treatment options for PE remain lacking (17). Current approaches for predicting PE occurrence lack sufficient sensitivity. Meanwhile, exosomes, known to transport miRNAs to distant organs, may play a crucial role in the systemic organ damage associated with PE and may serve as significant predictors of the condition (18). However, despite some studies reporting the potential endothelial regulatory role of exomiRs, they have not proven to be reliable predictors for PE, and their application poses a significant challenge (1). This review provides a comprehensive overview of the current evidence on exomiR function in PE and explores the potential utility of exomiRs in diagnosis, prediction, and treatment. By combining existing knowledge, this review enhances the understanding of PE pathogenesis and may contribute to the development of novel management strategies. We hope that this review will encourage more researchers to pay attention to the role of exomiRs as biomarkers, thereby contributing to the diagnosis and treatment of PE.
2 PE epigenetics
Waddington first used the term “epigenetics” in the 1940s to describe how an organism’s environment and genes might interact to cause non-Mendelian inheritance of phenotypes (19). This definition has evolved to “molecular factors and processes around DNA that regulate genome activity, independent of DNA sequence, and are mitotically stable” (20). Epigenetic modifications result from environmental changes that affect biological processes within an organism, affecting heritable variations in gene expression (21). The epigenetic disruption of gene expression patterns can lead to autoimmune disorders, cancers, and other diseases (22). DNA methylation, histone modifications, and non-coding RNAs (ncRNAs) are three basic epigenetic codes that have received considerable attention (23). Histone and DNA modifications play intermediate roles in controlling gene activity (24). Moreover, altering functional ncRNA regulation can modify gene activity, affecting chromatin structure, epigenetic memory, selective RNA splicing, and protein translation (24). Notably, gestational hypoxia permits adaptive reactions to modifications in the placental environment in preterm infants through epigenetics (25). In addition, PE and other pregnancy-related complications may be influenced by miRNAs belonging to ncRNAs (26). The overview of the epigenetic process in PE is shown in Figure 2.
2.1 DNA methylation during PE
DNA methylation, the most common epigenetic mechanism, involves the addition of a methyl group to cytosine-phospho-guanine (CpG) dinucleotide sites, catalyzed by DNA methyltransferases (DNMTs) (27). CpG sites are frequently located in genomic regions known as CpG islands, comprising nearly half of the human genome, and are frequently targeted by transcription factors (28). DNMT1 is responsible for maintaining genome-wide methylation, whereas DNMT3A and DNMT3B initiate de novo DNA methylation, establishing new methylation patterns (29). DNA methylation, serving as heritable epigenetic markers, is crucial to embryonic development, transcription, chromatin structure, and X-chromosome inactivation and can repress transcription or post-transcriptional RNA degradation to silence downstream target genes (25). Alterations in gene expression in the placentas of women with PE have been potentially attributed to epigenetic modifications induced by the abnormal placental environment (30). Further, most studies showing alterations in DNA methylation related to placental development gene expression in PE have reported direct correlations (31). Mayne et al. discovered 62 sites of abnormal DNA methylation in early PE linked to increased placental aging (32). Furthermore, Zadora et al. observed upregulation of the homeobox gene family, exhibiting diverse methylation patterns, including TLX1 and DLX5, which correlated with decreased trophoblast proliferation and PE (33). Additionally, Wang et al. reported elevated expression of vascular endothelial growth factor A (VEGFA), VEGFC, hypoxia-inducible factor 1-α (HIF1α), and RELA proto-oncogene associated with attenuation of the CpG island methylator phenotype (34). These findings suggest associations between PE and dysregulated angiogenic/anti-angiogenic factors, hypoxia, and placental insufficiency. Huang et al. postulated that adenosine, a crucial signaling molecule dysregulated in response to hypoxia, contributes to aberrant trophoblast invasion through epigenetic mechanisms involving DNA methylation modifications in specific tissues critical to PE pathophysiology (35). Simultaneously, increased placental DNA methylation of Wnt family member 2 and the metalloproteinase (MMP) promoter region, along with reduced methylation of placental tissue inhibitors of metalloproteinase 3 (TIMP3)—an MMP inhibitor—have been identified in PE. This may account for the loss of normal trophoblast invasion and spiral artery remodeling (36). Moreover, Anderson et al. demonstrated an association between DNA methylation and vitamin D metabolism, suggesting that vitamin D deficiency increases the risk of PE (37).
2.2 Histone modification during PE
Histone modifications, including posttranslational modifications such as histone methylation, acetylation, phosphorylation, and ubiquitination, are catalyzed by specific enzymes (38). Acetylation of histones H3 and H4 at specific lysine (K) and arginine (A) residues can modulate gene expression (39). Histone lysine methylation can cause either activation or inhibition, depending on its location (25). Eddy et al. observed a decrease in histone H3 acetylation in response to hypoxia in PE (40). Additionally, regulation of the histone demethylase Jumonji domain-containing 1A and histone deacetylation 2 (HDAC 2) is controlled in response to hypoxia (41). HIF1α is essential for controlling these hypoxia-regulated HDACs, which can exacerbate the PE phenotype by epigenetically altering the DNA packaging protein histone H3 and transactivating target genes (42). Moreover, normal trophoblast development requires interactions between HIF1α and HDAC (43). According to Wang et al., HDAC inhibition correlates with increased expression of chymase, a non-ACE angiotensin-converting enzyme. Chymase has been implicated in inflammation and vascular dysfunction and is upregulated in PE, implying that changes in HDAC expression may contribute to placental dysfunction in PE (44). Syncytin, a key regulator of syncytiotrophoblast (STB) formation, is regulated by the placenta-specific transcription factor and glial cells missing homolog 1 (GCMa). GCMa acetylation is modulated by the cAMP response element-binding protein to activate the cAMP/PKA pathway and induce trophoblast fusion (45). Furthermore, MMP and TIMP expression is regulated by histone H3 Lysine 9/29me3 (46).
2.3 ncRNAs
Accounting for 98% of the human genome, ncRNAs do not translate proteins (47) and can be divided into regulatory and housekeeping ncRNAs (48). The former includes miRNAs, long non-coding RNAs (lncRNAs), circular RNAs (circRNAs), piwi-interacting RNAs (piRNAs), small interfering RNAs (siRNAs), transfer RNAs, ribosomal RNAs, and small nuclear RNAs (49). Specifically, regulatory ncRNAs can modulate cellular activity through direct interactions (50). Meanwhile, myriad roles for miRNAs in placental growth have been reported, with their overexpression associated with pregnancy-related illnesses such as PE (51). Notably, differential lncRNA expression has been reported in the placenta and peripheral blood of healthy pregnant women compared to those with PE (52). Despite their implication in various disorders, circRNAs, piRNAs, and siRNAs have been relatively understudied in the context of PE pathophysiology (53).
Gene expression regulation in developing and differentiated tissues is facilitated by several epigenetic mechanisms, including DNA methylation, histone modification, and miRNA activity (21). These mechanisms influence gene expression during placental development and function (54) and may play critical roles in various pregnancy complications such as PE, gestational diabetes mellitus, fetal growth restriction, and preterm birth (55).
3 miRNAs and PE
Among ncRNAs, miRNAs—endogenous, short, single-stranded 20–24 nucleotide molecules—were discovered in the early 1990s (56). To date, miRNAs have been detected in nearly all plant and animal species, with more than 2000 identified in the human genome (57). Despite the absence of direct experimental evidence, computational studies have shown that nearly 60% of human genes are possible miRNA targets, suggesting a potential influence on all biological pathways (58). They primarily bind to the 3′ end of messenger RNA (mRNA) molecules and repress target mRNAs through transcript degradation, translation blockage, and gene expression suppression. This implies that miRNAs control target protein gene translation and mRNA degradation at the post-transcriptional level. Consequently, aberrant miRNA expression has been implicated in various malignancies, including ovarian, lung, and breast cancers, by modulating key cellular activities such as cell proliferation, differentiation, apoptosis, angiogenesis, and metabolism (59),.
Aberrant miRNAs can target downstream genes, leading to decreased trophoblast migration and invasion or enhanced cell death, contributing to PE (24). Certain abnormally expressed miRNAs aggregate in specific chromosomal areas to form closely linked clusters, including the chromosome 14 miRNA cluster (C14MC) and chromosome 19 miRNA cluster (C19MC) (60). C14MC comprises 52 miRNAs that regulate essential physiological processes, including immunological suppression, anti-inflammatory responses, and hypoxia-induced responses (61). Meanwhile, C19MC includes 46 miRNA genes detectable as early as 5 weeks of gestation (62). Overexpression of C19MC is associated with reduced migration in the EVT cell line HTR8/Svneo, suggesting that this cluster mediates decreased trophoblast migration, spiral artery remodeling, and placental ischemia in PE. Conversely, C14MC expression declines as pregnancy progresses (63). Pineles et al. first identified the overexpression of miR-210 and miR-182 in the placenta of patients with PE, representing new targets for PE pathogenesis (15). The first global transcriptome analysis of miRNAs conducted in 2009 using microarray technology revealed that 11 miRNAs were upregulated and 23 were downregulated in women with severe PE compared with controls (64). Differential miRNA expression in PE has also been associated with metabolic changes, transcriptional regulation, immune function, cardiovascular and reproductive development, cell cycle, cell adhesion, and relational signaling pathways, such as transforming growth factor β (TGF-β), Hippo, and mitogen-activated protein kinase signaling pathways, according to biological information analysis (13, 65). However, the roles of miRNAs in the pathophysiology of PE remain to be fully characterized. The investigation of miRNA functions and processes in PE is summarized in Figure 3.
3.1 Regulation of mesenchymal stem cell function
MSCs in the maternal decidua are central to controlling the pro-angiogenic, immunomodulatory, and anti-inflammatory milieu of the maternal–fetal interface during placentation (66). Alterations in decidual MSC cytokine production and miRNA expression have been observed in PE, with impaired survival, proliferation, and migration of MSCs isolated from women with PE (67, 68). Enhanced miR-181a expression in the umbilical cord and decidual-derived MSCs of patients with PE inhibits proliferation and reduces immunosuppressive qualities without affecting apoptosis (69). Furthermore, increased miR-494 levels in PE decidual MSCs inhibit the G1/S transition, influence proliferation by targeting cyclin D1 and cyclin-dependent kinase 6, and hinder M2 polarization of macrophages. Collectively, this causes an immune imbalance at the maternal–fetal interface mediated by decreased prostaglandin E2 secretion (70, 71). Conversely, overexpression of miR-495 accelerates MSC apoptosis and induces cellular senescence (72).
MSCs that show relative low immunogenicity can regenerate damaged tissue through both direct differentiation into tissue cells and indirect support by secreting cytokines to promote the proliferation of tissue cells (73). Furthermore, they can also be induced to adopt immunosuppressive phenotypes to inhibit inflammation and immune activation (74). What’s more, MSCs can facilitate angiogenesis to reconstruct the vascular network and restore blood supply in the placenta (75). MSCs can improve maternal-fetal outcomes in different animal models of PE by enhancing cell metabolism, combating OS, promoting a balance in angiogenesis, and anti-inflammation (66). Studies have demonstrated promising results in the application of MSCs to treat PE, indicating that MSCs could be considered a potential novel treatment option (76). Recent studies indicate that the pleiotropic effects of MSCs arise from the synthesis of soluble paracrine factors, rather than their ability to differentiate (77). Particularly, exosomes alone have been established in studies to be responsible for the therapeutic capabilities of MSCs in PE therapy (78). Mesenchymal stem cell-derived exosomes have the potential to delay the progression of PE and enhance outcomes by improving trophoblast function and placental angiogenesis, regulating immune responses, and reducing inflammation and OS (79).
3.2 Regulation of trophoblast function
Abnormally expressed miRNAs primarily regulate trophoblast invasion. Overexpression of miR-20a in PE placentas inhibits proliferation, migration, and invasion of the trophoblast cell line JEG-3 by suppressing forkhead box protein A1 mRNA and protein expression (80). Additionally, up-regulated miR-29b directly binds to the 3′ untranslated regions (UTRs) of myeloid cell leukemia sequence 1, MMP2, VEGFA, and integrin β1 (ITGβ1), inducing trophoblast apoptosis and inhibiting trophoblast invasion and angiogenesis in HTR-8/SVneo and BeWo cells (81). Meanwhile, miR-30a overexpression in JEG-3 cells reduces cell invasion, downregulates insulin-like growth factor 1 (IGF1) mRNA and protein expression, and promotes trophoblast apoptosis in PE (82). Notably, miR-29b and miR-30a are highly expressed in patients with PE (83). Additionally, miR-134, miR-181a, miR-299, and miR-675 inhibit trophoblast invasion and migration by targeting HDAC2, insulin-like growth factor 2, and ITGβ1, with increased circulating and placental levels in PE (84, 85). Conversely, miR-195, miR-376c, or miR-378a-5p promote the proliferation and invasion of HTR8/SVneo cells by targeting the TGF-β pathway components, including activin type II receptor, activin receptor-like kinase 5, and Nodal, with confirmed downregulation in circulating and placental levels in women with PE (84, 86). Gao et al. reported that miR-4421 overexpression in PE downregulates the aldosterone synthase gene (CYP11B2), inhibiting trophoblast proliferation and blocking cell cycle progression (83).
3.3 Regulation of placental angiogenesis
Several miRNAs may regulate the expression of angiogenesis-related factors in PE (87). Specifically, placental-specific miRNAs may be expressed by human villi trophoblasts, become encapsulated in exosomes, and subsequently released into the maternal circulation. This may partially explain how placental implantation defects contribute to systemic vascular dysfunction (88). Additionally, dysregulated miRNA expression in PE may affect the capillary formation capacity of umbilical vein endothelial cells (70). For example, the expression of miR-16, miR-29a, miR-144, miR-195-5p, miR-346, and miR-582-3p, which target VEGFA—a pro-angiogenic factor promoting vascular endothelial cell proliferation, blood vessel formation, and vascular permeability—is upregulated in PE (89, 90). Moreover, Wang et al. observed decreased viability and proliferative activity of decidua-derived mesenchymal stem cells upon miR-16 overexpression and increased viability in cells transfected with an anti-miR-16 antibody (91). Meanwhile, miR-17, miR-20a, and miR-20b are reportedly overexpressed in PE, potentially cooperating in the suppression of various angiogenesis-related genes, including Ephrin B2 (EFNB2), Ephrin type B receptor 4 (EPHB4), HIF1A, VEGFA, MMP2, TIMP2, TGFB, and interleukin-8 (IL8) (92, 93). In addition, EFNB2 and EPHB4 interact to regulate the internalization and signaling of VEGF receptor 2 (VEGFR2) and VEGFR3, mediating vascular cell adhesion, repulsion, and migration (94, 95). HIF1α, a transcription factor activated in response to hypoxia, regulates the expression of VEGFA, emphasizing its importance in placental remodeling during normal pregnancy and its potential role in PE pathogenesis. Additionally, MMP2 and TIMP2 are crucial in spiral artery remodeling during early gestation and in modulating the extracellular matrix during the initial angiogenic response (96, 97). Furthermore, Dicer silencing inhibits lef-7f and miR-27b expression, as well as capillary germination and endothelial cell tubule formation (98). In contrast, the downregulation of miR-126 is associated with pro-angiogenic traits in PE (99, 100). In summary, miR-126 regulates the VEGF pathway at various levels and is inversely related to the angiogenic characteristics of PE (101).
3.4 Regulation of placental renin–angiotensin system
Upregulation of anti-angiogenic factors in the placenta, such as soluble fms-like tyrosine-1 (sFlt-1) and soluble endoglin (sEng), contributes to aberrant placental vascularization (102, 103). Increased synthesis of sFlt-1 and endothelin is associated with enhanced development of an agonistic autoantibody against the angiotensin (ANG II) type 1 (AT1) receptor (AT1-AA) in PE (104, 105). Upregulation of AT1-AA correlates with higher blood pressure, elevated endothelin levels, and reduced levels of vasodilators, such as nitric oxide (NO) (106). Pregnant women become insensitive to ANG II-mediated vasoconstriction, maintaining normotension despite elevated renin, aldosterone, and ANG II levels (107). However, sensitivity to ANG II is reportedly enhanced in patients with PE (108). Particularly, the angiotensin II type 1 receptor (AT1R)-specific antibody, AT1-AA, plays a crucial role in PE pathophysiology (109). Notably, elevated levels of AT1-AAs in women with PE are associated with increased production of sFlt-1, sEng, IL-6, and endothelin, increased trophoblast apoptosis, and decreased VEGF expression (110, 111). Specific miRNAs also regulate AT1-AA biosynthesis (25). For instance, miR-155 downregulation correlates with increased AT1R expression (112). Moreover, upregulation of placental miRNA-181a and downregulation of miR-1301 in PE are associated with increased IL-6 production, leading to elevated AT1-AA levels (113, 114).
3.5 Regulation of placental NO production
NO, synthesized from L-arginine by nitric oxide synthase (NOS), is a key regulator of vascular resistance and hemodynamic changes during pregnancy (115). Choi et al. reported decreased NO and NOS levels are related to PE (116). In women with PE, the increased expression of miR-155, miR-221, and miR-222 has been linked to decreased NO production (15, 117). Moreover, overexpression of miR-155 in HUVECs is associated with decreased endothelial NOS (eNOS) expression by targeting the 3′ UTR of eNOS (118, 119).
In addition, Yang et al. discovered that miR-148a and miR-152 increase the expression of fatty acid-binding protein 4 in trophoblasts and increase lipid accumulation by regulating DNA methyltransferase 1, which is involved in abnormal lipid metabolism and inflammatory responses in PE pathogenesis (120). Furthermore, miR-1301 is downregulated in PE and may be involved in leptin control throughout pregnancy; however, it is inversely associated with maternal systolic and diastolic blood pressure before delivery (114).
4 Roles of exosomes in PE
Extracellular vesicles (EVs)—small semipermeable membrane vesicles—facilitate communication and interaction within or between tissues (121, 122). EVs that exist in myriad bodily fluids are categorized as exosomes, microvesicles, and apoptotic bodies according to the modes of biogenesis and release (123). They are excellent paracrine regulators of cellular crosstalk based on lipid bilayer membranes, DNAs, RNAs, proteins, and lipids (124). In the maternal-placental-fetal unit, microvesicles can be regarded as syncytiotrophoblast membrane microparticles (STBMs). Exosomes (30-150 nm) are microscopic particles formed by the inward folding of the plasma membrane and the creation of intracellular multivesicular bodies (125). STBMs are small vesicles (50-2000 nm) shed from the plasma membrane (126). Apoptotic bodies (500-4000 nm) are produced by apoptotic cells and are characterized by the presence of organelles within the vesicles (127). Exosomes, STBMs, and apoptotic bodies have a similar lipid bilayer membrane and carry genetic and protein cargo associated with the inflammatory reaction in PE (128). However, there are some subtle differences in their roles in PE. Exosomes are believed to have immunosuppressive functions because they can inhibit NK cytotoxicity, suppress T cell activity, and express immunomodulatory proteins such as HLA-G5 (129). Additionally, some studies suggest that exosomes containing miRNAs may have a pro-inflammatory effect by activating various inflammatory pathways in PE (88). Moreover, in vitro studies have shown that STBMs can adhere to T cells, B cells, and other immune cells, inducing an inflammatory response in PE (130). Unlike exosomes and STBMs, the target cells of apoptotic bodies are less variable, mainly including macrophages, dendritic cells, and other neighboring cells in PE. Typically in PE, macrophages tend to polarize towards the anti-inflammatory M2 phenotype after phagocytosing apoptotic bodies, and genetic information can be transferred during this process (131).
Particularly, exosomes are released through an endosome-dependent pathway, participate in cell-to-cell communication and are implicated in immune responses, viral pathogenesis, nervous system illness, cancer progression, and pregnancy (132, 133). Exosomes also act as mediators of fetal–maternal communication during implantation and placentation while modulating maternal responses, maintaining cellular metabolic homeostasis, promoting fetal vasculogenesis, and maternal uterine vascular adaptation (134, 135). The total number of circulating exosomes increases throughout gestation, along with pregnancy complications such as gestational diabetes (GDM) and PE (132, 136). The International Society for EVs recommends identifying specific exosome markers, such as CD81, CD9, and CD63 tetraspanins, through techniques like immunoelectron microscopy, flow cytometry, or Western blotting to confirm the exosomal origin of the retrieved vesicles in maternal circulation (137). Pregnancy-associated exosomes have been isolated from the blood of pregnant women at various gestational ages (138). Furthermore, the concentration and content of circulating exosomes may indicate changes in condition, metabolism, fetal growth, and maturation (139). Although the specific origin, payload, and roles of these exosomes in maternal circulation remain unclear, the primary source is the placenta, which releases exosomes into maternal circulation as early as 6 weeks into pregnancy (17). Placental disruption is proposed as a fundamental factor in PE development; therefore, an increased release of exosomes into maternal circulation by placental trophoblasts may be characteristic of this disorder (140).
Numerous humoral factors are involved in PE pathogenesis, characterized by chronic inflammation, leukocyte activation, and elevated blood cytokine levels (141). Increased tumor necrosis factor-α (TNF-α) levels in early pregnancy may induce the expression of intercellular adhesion molecule-1 (ICAM-1) on vascular endothelial cells (ECs) and trophoblasts, activating them within the context of chronic inflammation (142). Notably, soluble ICAM-1 can be released by leukocytes adhering to the vascular endothelium due to OS (143). Additionally, chronic inflammation activates lymphocyte function-associated antigen-1 in leukocytes, disrupting spiral artery remodeling with EC and trophoblast activation (144). These two EC activation pathways cause vascular dysfunction, contributing to PE. In these patients, immune tolerance to trophoblasts is maintained by the impaired interaction between human leukocyte antigen-G and decidual natural killer (NK) cells, dendritic cells, regulatory T (Treg) cells, and cytokines secreted by uterine NK cells in the decidua, resulting in EC and trophoblast dysfunction (145, 146). Subsequently, EVTs fail to adequately invade the uterine decidua and myometrium, resulting in impaired spiral artery remodeling. Type-1 T helper (Th1) cells and Th17 cells, which secrete proinflammatory cytokines including TNF-α, interferon-γ, IL-6, and IL-17, are also prominent in PE (140). Disruption of angiogenesis causes vascular dysfunction, contributing to poor placentation and PE pathogenesis (147). Increased levels of antivascular growth factors, including sFlt-1 and sEng, can decrease placental growth factor (PlGF) levels and reduce angiogenesis in the placenta (148). Exosomes reportedly transport various humoral factors, including miRNAs, to distant organs and may have important roles in PE (Figure 4).
4.1 ExomiRs in PE
Exosomes that contain miRNAs, known as exomiRs, can be absorbed by nearby or distant cells and are modified by receivers (149). In addition to their complex with argonaute (AGO) proteins, miRNAs are actively released by bundling in exosomes to avoid destruction by RNases (150, 151). Compared to AGO-bound miRNAs, a small portion of the total miRNA plasma population is made up of exomiRs, which are required for donor–receiver cell interactions and impact functional outcomes by modifying host gene expression in recipient cells (152, 153). As exomiRs are specifically packaged into exosomes and released by the cell to initiate cellular reprogramming, they have recently become important biomarkers for several illnesses (154, 155).
Placenta-derived exosomes contain abundant miRNAs that are regulated by various factors such as hypoxia, environmental factors, and epigenetic alterations (156). There are more than 600 exomiRs expressed in the placenta, which play critical roles in placental development and function by regulating the expression of genes involved in trophoblast proliferation, differentiation, invasion, migration, apoptosis, and angiogenesis (157). ExomiRs expression patterns in the placenta shift during gestation, for example, a comparison of placentas isolated from the first and third trimesters revealed a total of 208 miRNA transcripts that were differently expressed (158). In particular, exomiRs that regulate trophoblast proliferation and angiogenesis are significantly expressed in the first trimester, while exomiRs that promote trophoblast differentiation and reduce trophoblast proliferation are strongly expressed in the third trimester (158). Considering the upregulation of miRNAs in hypoxic cells and their long half-life and high stability, the relationships between miRNAs, including exomiRs, and PE have been extensively studied (159, 160). Different concentrations of exomiRs may be associated with the pathological state of PE (Table 1). Devor et al. demonstrated that women with PE and those with normal pregnancies exhibit different exomiR patterns during the first trimester, which may be exploited for early PE diagnosis (140). Moreover, exosomes secreted by cytotrophoblasts, which express placenta-specific miRNAs, participate in embryo implantation by promoting Treg cell differentiation and suppressing the nuclear factor-B signaling pathway, influencing the immune reaction and inflammatory response at the fetal–maternal interface and remodeling the spiral artery (122). ExomiRs, such as miR-517a, released from trophoblasts into the maternal circulation also have an important role in regulating the Th1/Th2 balance, leading to immune response activation, and their imbalance may contribute to PE pathogenesis (18). Notably, enrichment of specific exomiRs causes aberrant cell proliferation, adhesion, migration, and invasion in women with PE (177). Placental-specific miRNAs released into the maternal circulation by placental trophoblasts in exosomes may also impact TNF signaling regulation, which can induce vascular activation and dysfunction in placental trophoblasts by activating leukocytes and inducing vascular endothelial adhesion molecules (88). Moreover, the implanted embryo releases exomiRs to control blood flow (178).
Lower expression of miR23a-3p, miR-125b-2-3p, miR-144-3p, miR-192-5p, miR-205-5p, miR-208a-3p, miR-335-5p, miR-451a, miR-518a-3p, and miR-542-3p has been reported in exosomes isolated from patients with PE compared to normal controls, whereas the expression of let-7a-5p, miR-17-5p, miR-26a-5p, miR-30c-5p, miR-141-3p, miR-199a-3p, miR-210, miR-221-3p, miR-325-3p, miR-516-5p, miR-517, miR-520a, miR-525, miR-526a, miR-584-5p, miR-7445p, and miR-6724-5p is higher in patients with PE (121, 179). In addition to these exomiRs, miR-150 is a significant regulator of angiogenesis in utero that increases the expression of VEGF and Notch1. miR-150 expression is upregulated in umbilical cord mesenchymal stem cell-derived exosomes from a healthy pregnancy in piglets (165). Meanwhile, miR-153-3p is upregulated two-fold in exosomes from patients with PE. Its overexpression decreases cell proliferation and invasion while promoting apoptosis (8). Furthermore, miR-153-3p can bind the 3′ UTRs of HIF1A mRNA and inhibit its expression, which is associated with decreased tube formation by primary human umbilical vein ECs, decreased VEGFA production, and reduced angiogenesis (166, 169). Overexpression of exomiR-215-5p in PE reduces trophoblast proliferation and migration by decreasing cell division cycle 6 expression via the epigenetic downregulation of E-cadherin, which is required for DNA replication (167). Placental miR-342-3p, isolated from PE exosomes, has been implicated in EC dysfunction (180). ExomiRs, such as miR-486-1-5p and miR486-2-5p, participate in angiogenesis, migration, and placental development by targeting IGF1 (181). In contrast, hsa-miR-525-5p, hsa-miR-526b-5p, and hsa-miR-1269b are expressed exclusively under diseased conditions (182). Notably, hsa-miR-525-5p can decrease the expression of the anti-inflammatory factor vasoactive intestinal peptide (173). Meanwhile, hsa-miR-526b-5p modulates MMP1 and HIF1α expression (174), and hsa-miR-1269b regulates the expression of the forkhead box O1 gene (FOXO1), which is important for endometrial stromal decidualization and implantation (176).
4.2 Other functions of exosomes in PE
STB microvilli produced in the placenta may impede the proliferation and growth of ECs and serve as pathophysiological markers of PE (183). High levels of exosomes are released into the maternal circulation by STBs, causing endothelial dysfunction and leading to vascular constriction in PE (184). Exosomes may supply proteins to trophoblasts, generating a supportive environment that interferes with the operation of distant organs (18). Neprilysin (NEP), which may participate in PE pathogenesis by activating signaling peptides, such as endothelin and atrial natriuretic peptide, is widely expressed in placental trophoblasts and may directly promote impaired uteroplacental circulation (185, 186). Active NEP is reportedly secreted into the maternal circulation and coupled to STB-derived exosomes. Its expression is enhanced in PE, indicating that STB-derived exosomes could act as a bridge between placental dysfunction and subsequent clinical maternal disorders associated with PE (187). Additionally, various tissue factors are expressed on the surface of STB-derived exosomes, and its overexpression is related to PE (188). Moreover, exosomal syncytin-2 levels are significantly lower in the blood of patients with PE, which may be associated with STB production by villous trophoblasts (189). Furthermore, PE-derived exosomes participate in vascular dysfunction via their overexpression of sFlt-1 and sEng, which inhibit EC proliferation, migration, and differentiation, resulting in endothelial dysfunction (190). Notably, exosomes from PE may contribute to the spread of endothelial injury by sequestering free VEGF in maternal circulation (191).
5 Biomarker functions of exomiRs
Remarkably, the transfer of miRNAs by exosomes may result in the transmission of genetic information and generation of diverse proteins. ExomiRs are responsible for various physiological and pathological conditions in target cells and have been extensively investigated as biomarkers for the diagnosis, prediction, and treatment of many diseases, such as GDM and breast cancer (122).
Although multiple biomarkers, including sFlt-1, sEng, and PlGF, have been developed for PE diagnosis, their sensitivity and specificity are suboptimal due to the interference of many molecules. A reliable serum biomarker is required for an accurate and early diagnosis of PE. Molecular biomarkers, rather than biochemical indicators, may provide a more reliable platform for screening and diagnosing PE. The bioactivity, transfer, and, most importantly, uptake of exomiRs by target cells have been reported in pathological conditions associated with PE (192). For PE, exomiRs can be regarded as diagnostic biomarkers and therapeutic targets based on their differential expression levels (18, 122). Hence, detecting the expression of exomiRs and their gene products, such as exomiR-210-3p, may be useful for the diagnosis and prediction of PE. The ability of exosomes to transport vital information across cells can be exploited to improve clinical efficiency by leveraging their ability as biomarker candidates and prominent treatment targets (Figure 5). Recently, immune abnormalities in the placenta and maternal circulation have been found to occur before the clinical onset of PE (193). Particularly, Excessive systemic and placental complement activation, as well as poor adaptive T cell tolerance with Th1/Th2/Th17/Treg imbalance, have been observed in both human and animal models of PE (194). This indicates that the potential of immune-modifying therapy for preventing or treating PE is significant, despite the limited existing evidence (195). As mentioned above, exomiRs can regulate the Th1/Th2 balance and immune response, indicating that the regulation of exomiRs may help treat PE. Particularly, several studies have identified exomiR-15a-5p as a potential therapeutic target for PE due to its ability to inhibit trophoblast viability, migration, invasion, and epithelial-to-mesenchymal transition in trophoblasts (196).
Currently, there are no effective pharmaceutical treatments or strategies to prevent PE. Traditionally, exercise, low-dose aspirin and calcium intake are recommended to prevent PE in high-risk women by professional societies (197). Nowadays, statins, etanercept, sulfasalazine, hydroxychloroquine, eculizumab, metformin, and proton pump inhibitors are gaining more and more attention from researchers (9, 198). This review may provide a new idea for the prevention of PE. Targeting exomiRs and their related signaling pathways, transcripts, etc., may play a role in the prevention of PE. Research on PE is ongoing and will lead to innovative preventive methods as our knowledge of the condition progresses.
6 Conclusions and perspectives
The diagnosis, prediction, and treatment of PE are challenging owing to its complicated and heterogeneous nature. With the advancement of epigenetic research, distinct layers of the human placenta have been found to express hundreds of miRNAs associated with cell proliferation, differentiation, and apoptosis, implying an association between aberrant miRNA expression and PE. Specific miRNAs can influence MSC function by regulating their survival, proliferation, and migration. Moreover, several miRNAs are involved in the proliferation, migration, and invasion of trophoblasts, regulating their functions. In addition to controlling the expression of different factors related to angiogenesis, such as VEGFA, MMP2, and TIMP2, miRNAs contribute to the regulation of the placental renin–angiotensin system by controlling the expression of anti-angiogenic factors, such as sFlt-1 and sEng, associated with vascular resistance. Moreover, certain miRNAs regulate placental NO production and are linked to maternal blood pressure. In PE, exosomes may contain molecules with characteristics of damaged trophoblasts, including miRNAs and other proteins such as NEP and VEGF. The exosomal lipid bilayer is protected from the extracellular environment, making it an ideal delivery mechanism for miRNAs and endowing exomiRs with improved stability and protection from degradation compared with miRNAs.
In particular, exomiRs have the characteristics of disease-causing cells, and considering their significance and stability, they may represent an effective non-invasive method for the early diagnosis of PE in maternal peripheral blood. Additionally, exosomes in PE may include miRNAs with the characteristics of injured trophoblasts. Accordingly, analyzing exomiRs may aid in predicting the onset of PE. Moreover, exomiRs may be viable targets for treating PE. Since exomiRs can lead to the occurrence and development of PE through different pathways, inhibiting the expression of these exomiRs and blocking these pathways may be effective for treating PE. Specifically, measuring exomiRs, such as exomiR-210-3p and exomiR-520c-3p, may improve our ability to identify patients with PE earlier. Furthermore, varying levels of exomiR expression may be associated with the development and prognosis of PE, increasing the accuracy of predicting PE progression and clinical outcomes. That is, determining the concentration of exomiRs may assist in predicting the progression and prognosis of PE. Considering the overexpression and downregulation of exomiRs and their expression products, future PE treatments may involve targeting these exomiRs, specific signaling pathways, and downstream gene products, such as the PTEN/PI3K/AKT signaling pathway and IGF1.
In conclusion, the pathogenesis of PE has not been fully elucidated, and its early diagnosis, prevention, and treatment are significant but currently limited. The ability to identify exomiRs generated during pregnancy and implement non-invasive therapies to mitigate their effects may prove critical in clinical applications for the diagnosis, prediction, and treatment of PE. For example, it may be helpful for the diagnosis and prediction of PE by detecting the expression levels of exomiRs and transcripts. Additionally, targeting exomiRs and related pathways may have great potential for the treatment of PE. However, further research is warranted to identify the mechanisms underlying the release of exomiRs involved in PE progression.
Author contributions
YS: Writing – original draft. BH: Writing – original draft. JW: Writing – original draft. AC: Writing – review & editing. SL: Writing – review & editing.
Funding
The author(s) declare financial support was received for the research, authorship, and/or publication of this article. This work was supported by Natural Science Fund Project of Shandong Province (ZR2019MH127).
Acknowledgments
The authors thank Editage (www.editage.cn) for English language editing.
Conflict of interest
The authors declare that the research was conducted in the absence of any commercial or financial relationships that could be construed as a potential conflict of interest.
Publisher’s note
All claims expressed in this article are solely those of the authors and do not necessarily represent those of their affiliated organizations, or those of the publisher, the editors and the reviewers. Any product that may be evaluated in this article, or claim that may be made by its manufacturer, is not guaranteed or endorsed by the publisher.
References
1. Escudero CA, Herlitz K, Troncoso F, Acurio J, Aguayo C, Roberts JM, et al. Role of Extracellular Vesicles and microRNAs on Dysfunctional Angiogenesis during Preeclamptic Pregnancies. Front Physiol. (2016) 7:98. doi: 10.3389/fphys.2016.00098
2. Wang W, Xie X, Yuan T, Wang Y, Zhao F, Zhou Z, et al. Epidemiological trends of maternal hypertensive disorders of pregnancy at the global, regional, and national levels: a population-based study. BMC pregnancy childbirth. (2021) 21:364. doi: 10.1186/s12884-021-03809-2
3. MacDonald TM, Walker SP, Hannan NJ, Tong S, Kaitu’u-Lino TJ. Clinical tools and biomarkers to predict preeclampsia. EBioMedicine. (2022) 75:103780. doi: 10.1016/j.ebiom.2021.103780
4. Dimitriadis E, Rolnik DL, Zhou W, Estrada-Gutierrez G, Koga K, Francisco RPV, et al. Pre-eclampsia. Nat Rev Dis primers. (2023) 9:8. doi: 10.1038/s41572-023-00417-6
5. Deer E, Herrock O, Campbell N, Cornelius D, Fitzgerald S, Amaral LM, et al. The role of immune cells and mediators in preeclampsia. Nat Rev Nephrology. (2023) 19:257–70. doi: 10.1038/s41581-022-00670-0
6. Miller EC, Wilczek A, Bello NA, Tom S, Wapner R, Suh Y. Pregnancy, preeclampsia and maternal aging: From epidemiology to functional genomics. Ageing Res Rev. (2022) 73:101535. doi: 10.1016/j.arr.2021.101535
7. Rana S, Lemoine E, Granger JP, Karumanchi SA. Preeclampsia: pathophysiology, challenges, and perspectives. Circ Res. (2019) 124:1094–112. doi: 10.1161/circresaha.118.313276
8. Li H, Ouyang Y, Sadovsky E, Parks WT, Chu T, Sadovsky Y. Unique microRNA signals in plasma exosomes from pregnancies complicated by preeclampsia. Hypertension (Dallas Tex: 1979). (2020) 75:762–71. doi: 10.1161/hypertensionaha.119.14081
9. Ma’ayeh M, Costantine MM. Prevention of preeclampsia. Semin fetal neonatal Med. (2020) 25:101123. doi: 10.1016/j.siny.2020.101123
10. Grzeszczak K, Łanocha-Arendarczyk N, Malinowski W, Ziętek P, Kosik-Bogacka D. Oxidative stress in pregnancy. Biomolecules. (2023) 13:1768. doi: 10.3390/biom13121768
11. Chappell LC, Cluver CA, Kingdom J, Tong S. Pre-eclampsia. Lancet. (2021) 398:341–54. doi: 10.1016/s0140-6736(20)32335-7
12. Woo Kinshella ML, Sarr C, Sandhu A, Bone JN, Vidler M, Moore SE, et al. Calcium for pre-eclampsia prevention: A systematic review and network meta-analysis to guide personalised antenatal care. BJOG: an Int J obstetrics gynaecology. (2022) 129:1833–43. doi: 10.1111/1471-0528.17222
13. Lv Y, Lu C, Ji X, Miao Z, Long W, Ding H, et al. Roles of microRNAs in preeclampsia. J Cell Physiol. (2019) 234:1052–61. doi: 10.1002/jcp.27291
14. Maligianni I, Yapijakis C, Nousia K, Bacopoulou F, Chrousos GP. Exosomes and exosomal non-coding RNAs throughout human gestation (Review). Exp Ther Med. (2022) 24:582. doi: 10.3892/etm.2022.11518
15. Pineles BL, Romero R, Montenegro D, Tarca AL, Han YM, Kim YM, et al. Distinct subsets of microRNAs are expressed differentially in the human placentas of patients with preeclampsia. Am J obstetrics gynecology. (2007) 196:261. doi: 10.1016/j.ajog.2007.01.008
16. Tkachenko A, Illarionov R, Vashukova E, Glotov A. Publication-based analysis of miR-210 dependent biomarkers of pre-eclampsia. Biol Commun. (2020) 65:163–77. doi: 10.21638/spbu03.2020.203
17. Ghafourian M, Mahdavi R, Akbari Jonoush Z, Sadeghi M, Ghadiri N, Farzaneh M, et al. The implications of exosomes in pregnancy: emerging as new diagnostic markers and therapeutics targets. Cell communication signaling: CCS. (2022) 20:51. doi: 10.1186/s12964-022-00853-z
18. Matsubara K, Matsubara Y, Uchikura Y, Sugiyama T. Pathophysiology of preeclampsia: the role of exosomes. Int J Mol Sci. (2021) 22:2572. doi: 10.3390/ijms22052572
19. Van Speybroeck L. From epigenesis to epigenetics: the case of C. H. Waddington. Ann New York Acad Sci. (2002) 981:61–81. doi: 10.1111/j.1749-6632.2002.tb04912.x
20. Korolenko AA, Noll SE, Skinner MK. Epigenetic inheritance and transgenerational environmental justice. Yale J Biol Med. (2023) 96:241–50. doi: 10.59249/fkws5176
21. Andrawus M, Sharvit L, Atzmon G. Epigenetics and pregnancy: conditional snapshot or rolling event. Int J Mol Sci. (2022) 23:12698. doi: 10.3390/ijms232012698
22. Zhang L, Lu Q, Chang C. Epigenetics in health and disease. Adv Exp Med Biol. (2020) 1253:3–55. doi: 10.1007/978-981-15-3449-2_1
23. Boulias K, Greer EL. Biological roles of adenine methylation in RNA. Nat Rev Genet. (2023) 24:143–60. doi: 10.1038/s41576-022-00534-0
24. Sun N, Qin S, Zhang L, Liu S. Roles of noncoding RNAs in preeclampsia. Reprod Biol endocrinology: RB&E. (2021) 19:100. doi: 10.1186/s12958-021-00783-4
25. Ashraf UM, Hall DL, Rawls AZ, Alexander BT. Epigenetic processes during preeclampsia and effects on fetal development and chronic health. Clin Sci (London England: 1979). (2021) 135:2307–27. doi: 10.1042/cs20190070
26. Jiang Y, Luo T, Xia Q, Tian J, Yang J. microRNA-140-5p from human umbilical cord mesenchymal stem cells-released exosomes suppresses preeclampsia development. Funct Integr Genomics. (2022) 22:813–24. doi: 10.1007/s10142-022-00848-6
27. Mattei AL, Bailly N, Meissner A. DNA methylation: a historical perspective. Trends genetics: TIG. (2022) 38:676–707. doi: 10.1016/j.tig.2022.03.010
28. Deaton AM, Bird A. CpG islands and the regulation of transcription. Genes Dev. (2011) 25:1010–22. doi: 10.1101/gad.2037511
29. Badon SE, Littman AJ, Chan KCG, Tadesse MG, Stapleton PL, Bammler TK, et al. Physical activity and epigenetic biomarkers in maternal blood during pregnancy. Epigenomics. (2018) 10:1383–95. doi: 10.2217/epi-2017-0169
30. Aouache R, Biquard L, Vaiman D, Miralles F. Oxidative stress in preeclampsia and placental diseases. Int J Mol Sci. (2018) 19:1496. doi: 10.3390/ijms19051496
31. Almomani SN, Alsaleh AA, Weeks RJ, Chatterjee A, Day RC, Honda I, et al. Identification and validation of DNA methylation changes in pre-eclampsia. Placenta. (2021) 110:16–23. doi: 10.1016/j.placenta.2021.05.005
32. Mayne BT, Leemaqz SY, Smith AK, Breen J, Roberts CT, Bianco-Miotto T. Accelerated placental aging in early onset preeclampsia pregnancies identified by DNA methylation. Epigenomics. (2017) 9:279–89. doi: 10.2217/epi-2016-0103
33. Zadora J, Singh M, Herse F, Przybyl L, Haase N, Golic M, et al. Disturbed placental imprinting in preeclampsia leads to altered expression of DLX5, a human-specific early trophoblast marker. Circulation. (2017) 136:1824–39. doi: 10.1161/circulationaha.117.028110
34. Wang T, Xiang Y, Zhou X, Zheng X, Zhang H, Zhang X, et al. Epigenome-wide association data implicate fetal/maternal adaptations contributing to clinical outcomes in preeclampsia. Epigenomics. (2019) 11:1003–19. doi: 10.2217/epi-2019-0065
35. Huang A, Wu H, Iriyama T, Zhang Y, Sun K, Song A, et al. Elevated adenosine induces placental DNA hypomethylation independent of A2B receptor signaling in preeclampsia. Hypertension (Dallas Tex: 1979). (2017) 70:209–18. doi: 10.1161/hypertensionaha.117.09536
36. Li X, Wu C, Shen Y, Wang K, Tang L, Zhou M, et al. Ten-eleven translocation 2 demethylates the MMP9 promoter, and its down-regulation in preeclampsia impairs trophoblast migration and invasion. J Biol Chem. (2018) 293:10059–70. doi: 10.1074/jbc.RA117.001265
37. Anderson CM, Ralph JL, Johnson L, Scheett A, Wright ML, Taylor JY, et al. First trimester vitamin D status and placental epigenomics in preeclampsia among Northern Plains primiparas. Life Sci. (2015) 129:10–5. doi: 10.1016/j.lfs.2014.07.012
38. Zaib S, Rana N, Khan I. Histone modifications and their role in epigenetics of cancer. Curr medicinal Chem. (2022) 29:2399–411. doi: 10.2174/0929867328666211108105214
39. Rothbart SB, Strahl BD. Interpreting the language of histone and DNA modifications. Biochim Biophys Acta. (2014) 1839:627–43. doi: 10.1016/j.bbagrm.2014.03.001
40. Eddy AC, Chapman H, George EM. Acute hypoxia and chronic ischemia induce differential total changes in placental epigenetic modifications. Reprod Sci. (2019) 26:766–73. doi: 10.1177/1933719118799193
41. Wellmann S, Bettkober M, Zelmer A, Seeger K, Faigle M, Eltzschig HK, et al. Hypoxia upregulates the histone demethylase JMJD1A via HIF-1. Biochem Biophys Res Commun. (2008) 372:892–7. doi: 10.1016/j.bbrc.2008.05.150
42. Charron CE, Chou PC, Coutts DJC, Kumar V, To M, Akashi K, et al. Hypoxia-inducible factor 1alpha induces corticosteroid-insensitive inflammation via reduction of histone deacetylase-2 transcription. J Biol Chem. (2009) 284:36047–54. doi: 10.1074/jbc.M109.025387
43. Maltepe E, Krampitz GW, Okazaki KM, Red-Horse K, Mak W, Simon MC, et al. Hypoxia-inducible factor-dependent histone deacetylase activity determines stem cell fate in the placenta. Dev (Cambridge England). (2005) 132:3393–403. doi: 10.1242/dev.01923
44. Wang Y, Gu Y, Lewis DF, Alexander JS, Granger DN. Elevated plasma chymotrypsin-like protease (chymase) activity in women with preeclampsia. Hypertension pregnancy. (2010) 29:253–61. doi: 10.3109/10641950802001842
45. Chuang HC, Chang CW, Chang GD, Yao TP, Chen H. Histone deacetylase 3 binds to and regulates the GCMa transcription factor. Nucleic Acids Res. (2006) 34:1459–69. doi: 10.1093/nar/gkl048
46. Rahat B, Sharma R, Bagga R, Hamid A, Kaur J. Imbalance between matrix metalloproteinases and their tissue inhibitors in preeclampsia and gestational trophoblastic diseases. Reprod (Cambridge England). (2016) 152:11–22. doi: 10.1530/rep-16-0060
47. Panni S, Lovering RC, Porras P, Orchard S. Non-coding RNA regulatory networks. Biochim Biophys Acta Gene Regul mechanisms. (2020) 1863:194417. doi: 10.1016/j.bbagrm.2019.194417
48. Hemberg M, Gray JM, Cloonan N, Kuersten S, Grimmond S, Greenberg ME, et al. Integrated genome analysis suggests that most conserved non-coding sequences are regulatory factor binding sites. Nucleic Acids Res. (2012) 40:7858–69. doi: 10.1093/nar/gks477
49. Hombach S, Kretz M. Non-coding RNAs: classification, biology and functioning. Adv Exp Med Biol. (2016) 937:3–17. doi: 10.1007/978-3-319-42059-2_1
50. Anastasiadou E, Jacob LS, Slack FJ. Non-coding RNA networks in cancer. Nat Rev Cancer. (2018) 18:5–18. doi: 10.1038/nrc.2017.99
51. Fu G, Brkić J, Hayder H, Peng C. MicroRNAs in human placental development and pregnancy complications. Int J Mol Sci. (2013) 14:5519–44. doi: 10.3390/ijms14035519
52. Sun Q, Hao Q, Prasanth KV. Nuclear long noncoding RNAs: key regulators of gene expression. Trends genetics: TIG. (2018) 34:142–57. doi: 10.1016/j.tig.2017.11.005
53. Han B, Chao J, Yao H. Circular RNA and its mechanisms in disease: From the bench to the clinic. Pharmacol Ther. (2018) 187:31–44. doi: 10.1016/j.pharmthera.2018.01.010
54. Best JD, Carey N. The epigenetics of normal pregnancy. Obstetric Med. (2013) 6:3–7. doi: 10.1258/om.2011.110070
55. Meng M, Cheng YKY, Wu L, Chaemsaithong P, Leung MBW, Chim SSC, et al. Whole genome miRNA profiling revealed miR-199a as potential placental pathogenesis of selective fetal growth restriction in monochorionic twin pregnancies. Placenta. (2020) 92:44–53. doi: 10.1016/j.placenta.2020.02.002
56. Shukla GC, Singh J, Barik S. MicroRNAs: processing, maturation, target recognition and regulatory functions. Mol Cell Pharmacol. (2011) 3:83–92.
57. Hill M, Tran N. miRNA interplay: mechanisms and consequences in cancer. Dis Models mechanisms. (2021) 14:dmm047662. doi: 10.1242/dmm.047662
58. Li JY, Yong TY, Michael MZ, Gleadle JM. MicroRNAs: are they the missing link between hypoxia and pre-eclampsia? Hypertension pregnancy. (2014) 33:102–14. doi: 10.3109/10641955.2013.832772
59. Pan HT, Shi XL, Fang M, Sun XM, Chen PP, Ding JL, et al. Profiling of exosomal microRNAs expression in umbilical cord blood from normal and preeclampsia patients. BMC pregnancy childbirth. (2022) 22:124. doi: 10.1186/s12884-022-04449-w
60. Donker RB, Mouillet JF, Chu T, Hubel CA, Stolz DB, Morelli AE, et al. The expression profile of C19MC microRNAs in primary human trophoblast cells and exosomes. Mol Hum reproduction. (2012) 18:417–24. doi: 10.1093/molehr/gas013
61. Morales-Prieto DM, Ospina-Prieto S, Chaiwangyen W, Schoenleben M, Markert UR. Pregnancy-associated miRNA-clusters. J Reprod Immunol. (2013) 97:51–61. doi: 10.1016/j.jri.2012.11.001
62. Flor I, Neumann A, Freter C, Helmke BM, Langenbuch M, Rippe V, et al. Abundant expression and hemimethylation of C19MC in cell cultures from placenta-derived stromal cells. Biochem Biophys Res Commun. (2012) 422:411–6. doi: 10.1016/j.bbrc.2012.05.004
63. Liang Y, Ridzon D, Wong L, Chen C. Characterization of microRNA expression profiles in normal human tissues. BMC Genomics. (2007) 8:166. doi: 10.1186/1471-2164-8-166
64. Zhu XM, Han T, Sargent IL, Yin GW, Yao YQ. Differential expression profile of microRNAs in human placentas from preeclamptic pregnancies vs normal pregnancies. Am J obstetrics gynecology. (2009) 200:661. doi: 10.1016/j.ajog.2008.12.045
65. Luo S, Cao N, Tang Y, Gu W. Identification of key microRNAs and genes in preeclampsia by bioinformatics analysis. PloS One. (2017) 12:e0178549. doi: 10.1371/journal.pone.0178549
66. Suvakov S, Richards C, Nikolic V, Simic T, McGrath K, Krasnodembskaya A, et al. Emerging therapeutic potential of mesenchymal stem/stromal cells in preeclampsia. Curr hypertension Rep. (2020) 22:37. doi: 10.1007/s11906-020-1034-8
67. Zhao G, Zhou X, Chen S, Miao H, Fan H, Wang Z, et al. Differential expression of microRNAs in decidua-derived mesenchymal stem cells from patients with pre-eclampsia. J Biomed science. (2014) 21:81. doi: 10.1186/s12929-014-0081-3
68. Kusuma GD, Georgiou HM, Perkins AV, Abumaree MH, Brennecke SP, Kalionis B. Mesenchymal stem/stromal cells and their role in oxidative stress associated with preeclampsia. Yale J Biol Med. (2022) 95:115–27.
69. Liu L, Wang Y, Fan H, Zhao X, Liu D, Hu Y, et al. MicroRNA-181a regulates local immune balance by inhibiting proliferation and immunosuppressive properties of mesenchymal stem cells. Stem Cells (Dayton Ohio). (2012) 30:1756–70. doi: 10.1002/stem.1156
70. Chen S, Zhao G, Miao H, Tang R, Song Y, Hu Y, et al. MicroRNA-494 inhibits the growth and angiogenesis-regulating potential of mesenchymal stem cells. FEBS letters. (2015) 589:710–7. doi: 10.1016/j.febslet.2015.01.038
71. Zhao G, Miao H, Li X, Chen S, Hu Y, Wang Z, et al. TGF-β3-induced miR-494 inhibits macrophage polarization via suppressing PGE2 secretion in mesenchymal stem cells. FEBS letters. (2016) 590:1602–13. doi: 10.1002/1873-3468.12200
72. Li X, Song Y, Liu D, Zhao J, Xu J, Ren J, et al. MiR-495 promotes senescence of mesenchymal stem cells by targeting bmi-1. Cell Physiol Biochem. (2017) 42:780–96. doi: 10.1159/000478069
73. Jiang W, Xu J. Immune modulation by mesenchymal stem cells. Cell proliferation. (2020) 53:e12712. doi: 10.1111/cpr.12712
74. Gorodetsky R, Aicher WK. Allogenic use of human placenta-derived stromal cells as a highly active subtype of mesenchymal stromal cells for cell-based therapies. Int J Mol Sci. (2021) 22:5302. doi: 10.3390/ijms22105302
75. Jin S, Wu C, Chen M, Sun D, Zhang H. The pathological and therapeutic roles of mesenchymal stem cells in preeclampsia. Front Med. (2022) 9:923334. doi: 10.3389/fmed.2022.923334
76. Chang X, He Q, Wei M, Jia L, Wei Y, Bian Y, et al. Human umbilical cord mesenchymal stem cell derived exosomes (HUCMSC-exos) recovery soluble fms-like tyrosine kinase-1 (sFlt-1)-induced endothelial dysfunction in preeclampsia. Eur J Med Res. (2023) 28:277. doi: 10.1186/s40001-023-01182-8
77. Wang D, Na Q, Song GY, Wang L. Human umbilical cord mesenchymal stem cell-derived exosome-mediated transfer of microRNA-133b boosts trophoblast cell proliferation, migration and invasion in preeclampsia by restricting SGK1. Cell Cycle (Georgetown Tex). (2020) 19:1869–83. doi: 10.1080/15384101.2020.1769394
78. Margiana R. Mesenchymal stem cell-derived exosomes in preeclampsia: A next-generation therapeutic tool. Cell Biochem Funct. (2024) 42:e3908. doi: 10.1002/cbf.3908
79. Shi H, Yang Z, Cui J, Tao H, Ma R, Zhao Y. Mesenchymal stem cell-derived exosomes: a promising alternative in the therapy of preeclampsia. Stem Cell Res Ther. (2024) 15:30. doi: 10.1186/s13287-024-03652-0
80. Wang Y, Zhang Y, Wang H, Wang J, Zhang Y, Wang Y, et al. Aberrantly up-regulated miR-20a in pre-eclampsic placenta compromised the proliferative and invasive behaviors of trophoblast cells by targeting forkhead box protein A1. Int J Biol Sci. (2014) 10:973–82. doi: 10.7150/ijbs.9088
81. Li P, Guo W, Du L, Zhao J, Wang Y, Liu L, et al. microRNA-29b contributes to pre-eclampsia through its effects on apoptosis, invasion and angiogenesis of trophoblast cells. Clin Sci (London England: 1979). (2013) 124:27–40. doi: 10.1042/cs20120121
82. Niu ZR, Han T, Sun XL, Luan LX, Gou WL, Zhu XM. MicroRNA-30a-3p is overexpressed in the placentas of patients with preeclampsia and affects trophoblast invasion and apoptosis by its effects on IGF-1. Am J obstetrics gynecology. (2018) 218:249.e1–.e12. doi: 10.1016/j.ajog.2017.11.568
83. Gao X, Li H, Wei JX. MiR-4421 regulates the progression of preeclampsia by regulating CYP11B2. Eur Rev Med Pharmacol Sci. (2018) 22:1533–40. doi: 10.26355/eurrev_201803_14557
84. Gunel T, Kamali N, Hosseini MK, Gumusoglu E, Benian A, Aydinli K. Regulatory effect of miR-195 in the placental dysfunction of preeclampsia. J maternal-fetal neonatal Med. (2020) 33:901–8. doi: 10.1080/14767058.2018.1508439
85. Wu L, Song WY, Xie Y, Hu LL, Hou XM, Wang R, et al. miR-181a-5p suppresses invasion and migration of HTR-8/SVneo cells by directly targeting IGF2BP2. Cell Death disease. (2018) 9:16. doi: 10.1038/s41419-017-0045-0
86. Fu G, Ye G, Nadeem L, Ji L, Manchanda T, Wang Y, et al. MicroRNA-376c impairs transforming growth factor-β and nodal signaling to promote trophoblast cell proliferation and invasion. Hypertension (Dallas Tex: 1979). (2013) 61:864–72. doi: 10.1161/hypertensionaha.111.203489
87. Reynolds LP, Borowicz PP, Caton JS, Vonnahme KA, Luther JS, Buchanan DS, et al. Uteroplacental vascular development and placental function: an update. Int J Dev Biol. (2010) 54:355–66. doi: 10.1387/ijdb.082799lr
88. Luo SS, Ishibashi O, Ishikawa G, Ishikawa T, Katayama A, Mishima T, et al. Human villous trophoblasts express and secrete placenta-specific microRNAs into maternal circulation via exosomes. Biol reproduction. (2009) 81:717–29. doi: 10.1095/biolreprod.108.075481
89. Akgör U, Ayaz L, Çayan F. Expression levels of maternal plasma microRNAs in preeclamptic pregnancies. J obstetrics gynaecology. (2021) 41:910–4. doi: 10.1080/01443615.2020.1820465
90. Boldeanu L, Dijmărescu AL, Radu M, Siloşi CA, Popescu-Drigă MV, Poenariu IS, et al. The role of mediating factors involved in angiogenesis during implantation. Romanian J morphology embryology = Rev roumaine morphologie embryologie. (2020) 61:665–72. doi: 10.47162/rjme.61.3.04
91. Wang Y, Fan H, Zhao G, Liu D, Du L, Wang Z, et al. miR-16 inhibits the proliferation and angiogenesis-regulating potential of mesenchymal stem cells in severe pre-eclampsia. FEBS J. (2012) 279:4510–24. doi: 10.1111/febs.12037
92. Wang W, Feng L, Zhang H, Hachy S, Satohisa S, Laurent LC, et al. Preeclampsia up-regulates angiogenesis-associated microRNA (i.e., miR-17, -20a, and -20b) that target ephrin-B2 and EPHB4 in human placenta. J Clin Endocrinol Metab. (2012) 97:E1051–9. doi: 10.1210/jc.2011-3131
93. Dews M, Fox JL, Hultine S, Sundaram P, Wang W, Liu YY, et al. The myc-miR-17~92 axis blunts TGF{beta} signaling and production of multiple TGF{beta}-dependent antiangiogenic factors. Cancer Res. (2010) 70:8233–46. doi: 10.1158/0008-5472.Can-10-2412
94. Mosch B, Reissenweber B, Neuber C, Pietzsch J. Eph receptors and ephrin ligands: important players in angiogenesis and tumor angiogenesis. J Oncol. (2010) 2010:135285. doi: 10.1155/2010/135285
95. Steinle JJ, Meininger CJ, Forough R, Wu G, Wu MH, Granger HJ. Eph B4 receptor signaling mediates endothelial cell migration and proliferation via the phosphatidylinositol 3-kinase pathway. J Biol Chem. (2002) 277:43830–5. doi: 10.1074/jbc.M207221200
96. Lockwood CJ, Oner C, Uz YH, Kayisli UA, Huang SJ, Buchwalder LF, et al. Matrix metalloproteinase 9 (MMP9) expression in preeclamptic decidua and MMP9 induction by tumor necrosis factor alpha and interleukin 1 beta in human first trimester decidual cells. Biol reproduction. (2008) 78:1064–72. doi: 10.1095/biolreprod.107.063743
97. Naruse K, Lash GE, Innes BA, Otun HA, Searle RF, Robson SC, et al. Localization of matrix metalloproteinase (MMP)-2, MMP-9 and tissue inhibitors for MMPs (TIMPs) in uterine natural killer cells in early human pregnancy. Hum Reprod (Oxford England). (2009) 24:553–61. doi: 10.1093/humrep/den408
98. Kuehbacher A, Urbich C, Zeiher AM, Dimmeler S. Role of Dicer and Drosha for endothelial microRNA expression and angiogenesis. Circ Res. (2007) 101:59–68. doi: 10.1161/circresaha.107.153916
99. Yan T, Liu Y, Cui K, Hu B, Wang F, Zou L. MicroRNA-126 regulates EPCs function: implications for a role of miR-126 in preeclampsia. J Cell Biochem. (2013) 114:2148–59. doi: 10.1002/jcb.24563
100. Dong A, Shen J, Zeng M, Campochiaro PA. Vascular cell-adhesion molecule-1 plays a central role in the proangiogenic effects of oxidative stress. Proc Natl Acad Sci United States America. (2011) 108:14614–9. doi: 10.1073/pnas.1012859108
101. Hong F, Li Y, Xu Y. Decreased placental miR-126 expression and vascular endothelial growth factor levels in patients with pre-eclampsia. J Int Med Res. (2014) 42:1243–51. doi: 10.1177/0300060514540627
102. Chaiworapongsa T, Romero R, Kim YM, Kim GJ, Kim MR, Espinoza J, et al. Plasma soluble vascular endothelial growth factor receptor-1 concentration is elevated prior to the clinical diagnosis of pre-eclampsia. J maternal-fetal neonatal Med. (2005) 17:3–18. doi: 10.1080/14767050400028816
103. Leaños-Miranda A, Navarro-Romero CS, Sillas-Pardo LJ, Ramírez-Valenzuela KL, Isordia-Salas I, Jiménez-Trejo LM. Soluble endoglin as a marker for preeclampsia, its severity, and the occurrence of adverse outcomes. Hypertension (Dallas Tex: 1979). (2019) 74:991–7. doi: 10.1161/hypertensionaha.119.13348
104. Suzuki H, Hirashima C, Nagayama S, Takahashi K, Yamamoto T, Matsubara S, et al. Increased serum levels of sFlt-1/PlGF ratio in preeclamptic women with onset at <32 weeks compared with ≥32 weeks. Pregnancy hypertension. (2018) 12:96–103. doi: 10.1016/j.preghy.2018.03.008
105. Lu YP, Hasan AA, Zeng S, Hocher B. Plasma ET-1 concentrations are elevated in pregnant women with hypertension -meta-analysis of clinical studies. Kidney Blood Pressure Res. (2017) 42:654–63. doi: 10.1159/000482004
106. Cunningham MW Jr., Castillo J, Ibrahim T, Cornelius DC, Campbell N, Amaral L, et al. AT1-AA (Angiotensin II type 1 receptor agonistic autoantibody) blockade prevents preeclamptic symptoms in placental ischemic rats. Hypertension (Dallas Tex: 1979). (2018) 71:886–93. doi: 10.1161/hypertensionaha.117.10681
107. Shah DM. Role of the renin-angiotensin system in the pathogenesis of preeclampsia. Am J Physiol Renal Physiol. (2005) 288:F614–25. doi: 10.1152/ajprenal.00410.2003
108. Brosnihan KB, Merrill DC, Yamaleyeva LM, Chen K, Neves L, Joyner J, et al. Longitudinal study of angiotensin peptides in normal and pre-eclamptic pregnancy. Endocrine. (2020) 69:410–9. doi: 10.1007/s12020-020-02296-3
109. Zhou CC, Zhang Y, Irani RA, Zhang H, Mi T, Popek EJ, et al. Angiotensin receptor agonistic autoantibodies induce pre-eclampsia in pregnant mice. Nat Med. (2008) 14:855–62. doi: 10.1038/nm.1856
110. Irani RA, Zhang Y, Blackwell SC, Zhou CC, Ramin SM, Kellems RE, et al. The detrimental role of angiotensin receptor agonistic autoantibodies in intrauterine growth restriction seen in preeclampsia. J Exp Med. (2009) 206:2809–22. doi: 10.1084/jem.20090872
111. Zhou CC, Irani RA, Dai Y, Blackwell SC, Hicks MJ, Ramin SM, et al. Autoantibody-mediated IL-6-dependent endothelin-1 elevation underlies pathogenesis in a mouse model of preeclampsia. J Immunol (Baltimore Md: 1950). (2011) 186:6024–34. doi: 10.4049/jimmunol.1004026
112. Teng G, Papavasiliou FN. Shhh! Silencing by microRNA-155. Philos Trans R Soc London Ser B Biol Sci. (2009) 364:631–7. doi: 10.1098/rstb.2008.0209
113. Cornelius DC, Amaral LM, Harmon A, Wallace K, Thomas AJ, Campbell N, et al. An increased population of regulatory T cells improves the pathophysiology of placental ischemia in a rat model of preeclampsia. Am J Physiol Regulatory Integr Comp Physiol. (2015) 309:R884–91. doi: 10.1152/ajpregu.00154.2015
114. Weedon-Fekjær MS, Sheng Y, Sugulle M, Johnsen GM, Herse F, Redman CW, et al. Placental miR-1301 is dysregulated in early-onset preeclampsia and inversely correlated with maternal circulating leptin. Placenta. (2014) 35:709–17. doi: 10.1016/j.placenta.2014.07.002
115. Guerby P, Tasta O, Swiader A, Pont F, Bujold E, Parant O, et al. Role of oxidative stress in the dysfunction of the placental endothelial nitric oxide synthase in preeclampsia. Redox Biol. (2021) 40:101861. doi: 10.1016/j.redox.2021.101861
116. Choi JW, Im MW, Pai SH. Nitric oxide production increases during normal pregnancy and decreases in preeclampsia. Ann Clin Lab science. (2002) 32:257–63.
117. Hu Y, Li P, Hao S, Liu L, Zhao J, Hou Y. Differential expression of microRNAs in the placentae of Chinese patients with severe pre-eclampsia. Clin Chem Lab Med. (2009) 47:923–9. doi: 10.1515/cclm.2009.228
118. Shen L, Li Y, Li R, Diao Z, Yany M, Wu M, et al. Placenta−associated serum exosomal miR−155 derived from patients with preeclampsia inhibits eNOS expression in human umbilical vein endothelial cells. Int J Mol Med. (2018) 41:1731–9. doi: 10.3892/ijmm.2018.3367
119. Kim J, Lee KS, Kim JH, Lee DK, Park M, Choi S, et al. Aspirin prevents TNF-α-induced endothelial cell dysfunction by regulating the NF-κB-dependent miR-155/eNOS pathway: Role of a miR-155/eNOS axis in preeclampsia. Free Radical Biol Med. (2017) 104:185–98. doi: 10.1016/j.freeradbiomed.2017.01.010
120. Yang A, Zhang H, Sun Y, Wang Y, Yang X, Yang X, et al. Modulation of FABP4 hypomethylation by DNMT1 and its inverse interaction with miR-148a/152 in the placenta of preeclamptic rats and HTR-8 cells. Placenta. (2016) 46:49–62. doi: 10.1016/j.placenta.2016.08.086
121. Esfandyari S, Elkafas H, Chugh RM, Park HS, Navarro A, Al-Hendy A. Exosomes as biomarkers for female reproductive diseases diagnosis and therapy. Int J Mol Sci. (2021) 22:2165. doi: 10.3390/ijms22042165
122. Czernek L, Düchler M. Exosomes as messengers between mother and fetus in pregnancy. Int J Mol Sci. (2020) 21:4264. doi: 10.3390/ijms21124264
123. Konečná B, Tóthová Ľ, Repiská G. Exosomes-associated DNA-new marker in pregnancy complications? Int J Mol Sci. (2019) 20:2890. doi: 10.3390/ijms20122890
124. Rezaie J, Ajezi S, Avci ÇB, Karimipour M, Geranmayeh MH, Nourazarian A, et al. Exosomes and their application in biomedical field: difficulties and advantages. Mol neurobiology. (2018) 55:3372–93. doi: 10.1007/s12035-017-0582-7
125. Pan BT, Teng K, Wu C, Adam M, Johnstone RM. Electron microscopic evidence for externalization of the transferrin receptor in vesicular form in sheep reticulocytes. J Cell Biol. (1985) 101:942–8. doi: 10.1083/jcb.101.3.942
126. Ståhl AL, Johansson K, Mossberg M, Kahn R, Karpman D. Exosomes and microvesicles in normal physiology, pathophysiology, and renal diseases. Pediatr Nephrol (Berlin Germany). (2019) 34:11–30. doi: 10.1007/s00467-017-3816-z
127. Gao P, Zhou L, Wu J, Weng W, Wang H, Ye M, et al. Riding apoptotic bodies for cell-cell transmission by African swine fever virus. Proc Natl Acad Sci United States America. (2023) 120:e2309506120. doi: 10.1073/pnas.2309506120
128. Wen J, Creaven D, Luan X, Wang J. Comparison of immunotherapy mediated by apoptotic bodies, microvesicles and exosomes: apoptotic bodies’ unique anti-inflammatory potential. J Trans Med. (2023) 21:478. doi: 10.1186/s12967-023-04342-w
129. Kshirsagar SK, Alam SM, Jasti S, Hodes H, Nauser T, Gilliam M, et al. Immunomodulatory molecules are released from the first trimester and term placenta via exosomes. Placenta. (2012) 33:982–90. doi: 10.1016/j.placenta.2012.10.005
130. Messerli M, May K, Hansson SR, Schneider H, Holzgreve W, Hahn S, et al. Feto-maternal interactions in pregnancies: placental microparticles activate peripheral blood monocytes. Placenta. (2010) 31:106–12. doi: 10.1016/j.placenta.2009.11.011
131. Akers JC, Gonda D, Kim R, Carter BS, Chen CC. Biogenesis of extracellular vesicles (EV): exosomes, microvesicles, retrovirus-like vesicles, and apoptotic bodies. J neuro-oncology. (2013) 113:1–11. doi: 10.1007/s11060-013-1084-8
132. Salomon C, Guanzon D, Scholz-Romero K, Longo S, Correa P, Illanes SE, et al. Placental exosomes as early biomarker of preeclampsia: potential role of exosomal microRNAs across gestation. J Clin Endocrinol Metab. (2017) 102:3182–94. doi: 10.1210/jc.2017-00672
133. Bai K, Li X, Zhong J, Ng EHY, Yeung WSB, Lee CL, et al. Placenta-derived exosomes as a modulator in maternal immune tolerance during pregnancy. Front Immunol. (2021) 12:671093. doi: 10.3389/fimmu.2021.671093
134. Han C, Wang C, Chen Y, Wang J, Xu X, Hilton T, et al. Placenta-derived extracellular vesicles induce preeclampsia in mouse models. Haematologica. (2020) 105:1686–94. doi: 10.3324/haematol.2019.226209
135. Hromadnikova I, Dvorakova L, Kotlabova K, Krofta L. The Prediction of Gestational Hypertension, Preeclampsia and Fetal Growth Restriction via the First Trimester Screening of Plasma Exosomal C19MC microRNAs. Int J Mol Sci. (2019) 20:2972. doi: 10.3390/ijms20122972
136. Sarker S, Scholz-Romero K, Perez A, Illanes SE, Mitchell MD, Rice GE, et al. Placenta-derived exosomes continuously increase in maternal circulation over the first trimester of pregnancy. J Trans Med. (2014) 12:204. doi: 10.1186/1479-5876-12-204
137. Burkova EE, Sedykh SE, Nevinsky GA. Human placenta exosomes: biogenesis, isolation, composition, and prospects for use in diagnostics. Int J Mol Sci. (2021) 22:2158. doi: 10.3390/ijms22042158
138. Paul N, Sultana Z, Fisher JJ, Maiti K, Smith R. Extracellular vesicles- crucial players in human pregnancy. Placenta. (2023) 140:30–8. doi: 10.1016/j.placenta.2023.07.006
139. Zhang J, Li H, Fan B, Xu W, Zhang X. Extracellular vesicles in normal pregnancy and pregnancy-related diseases. J Cell Mol Med. (2020) 24:4377–88. doi: 10.1111/jcmm.15144
140. Saito S, Sakai M. Th1/Th2 balance in preeclampsia. J Reprod Immunol. (2003) 59:161–73. doi: 10.1016/s0165-0378(03)00045-7
141. Abe E, Matsubara K, Oka K, Kusanagi Y, Ito M. Cytokine regulation of intercellular adhesion molecule-1 expression on trophoblasts in preeclampsia. Gynecologic obstetric Invest. (2008) 66:27–33. doi: 10.1159/000114253
142. Ballegeer VC, Spitz B, De Baene LA, Van Assche AF, Hidajat M, Criel AM. Platelet activation and vascular damage in gestational hypertension. Am J obstetrics gynecology. (1992) 166:629–33. doi: 10.1016/0002-9378(92)91689-8
143. Zhao J, Zheng DY, Yang JM, Wang M, Zhang XT, Sun L, et al. Maternal serum uric acid concentration is associated with the expression of tumour necrosis factor-α and intercellular adhesion molecule-1 in patients with preeclampsia. J Hum hypertension. (2016) 30:456–62. doi: 10.1038/jhh.2015.110
144. Yanagisawa M, Kurihara H, Kimura S, Tomobe Y, Kobayashi M, Mitsui Y, et al. A novel potent vasoconstrictor peptide produced by vascular endothelial cells. Nature. (1988) 332:411–5. doi: 10.1038/332411a0
145. Murata H, Tanaka S, Tsuzuki-Nakao T, Kido T, Kakita-Kobayashi M, Kida N, et al. The transcription factor HAND2 up-regulates transcription of the IL15 gene in human endometrial stromal cells. J Biol Chem. (2020) 295:9596–605. doi: 10.1074/jbc.RA120.012753
146. Xu X, Zhou Y, Wei H. Roles of HLA-G in the maternal-fetal immune microenvironment. Front Immunol. (2020) 11:592010. doi: 10.3389/fimmu.2020.592010
147. Lam C, Lim KH, Karumanchi SA. Circulating angiogenic factors in the pathogenesis and prediction of preeclampsia. Hypertension (Dallas Tex: 1979). (2005) 46:1077–85. doi: 10.1161/01.HYP.0000187899.34379.b0
148. Levine RJ, Maynard SE, Qian C, Lim KH, England LJ, Yu KF, et al. Circulating angiogenic factors and the risk of preeclampsia. N Engl J Med. (2004) 350:672–83. doi: 10.1056/NEJMoa031884
149. Murugesan S, Saravanakumar L, Powell MF, Rajasekaran NS, Kannappan R, Berkowitz DE. Role of exosomal microRNA signatures: An emerging factor in preeclampsia-mediated cardiovascular disease. Placenta. (2021) 103:226–31. doi: 10.1016/j.placenta.2020.10.033
150. Arroyo JD, Chevillet JR, Kroh EM, Ruf IK, Pritchard CC, Gibson DF, et al. Argonaute2 complexes carry a population of circulating microRNAs independent of vesicles in human plasma. Proc Natl Acad Sci United States America. (2011) 108:5003–8. doi: 10.1073/pnas.1019055108
151. Chen X, Liang H, Zhang J, Zen K, Zhang CY. Secreted microRNAs: a new form of intercellular communication. Trends Cell Biol. (2012) 22:125–32. doi: 10.1016/j.tcb.2011.12.001
152. Valadi H, Ekström K, Bossios A, Sjöstrand M, Lee JJ, Lötvall JO. Exosome-mediated transfer of mRNAs and microRNAs is a novel mechanism of genetic exchange between cells. Nat Cell Biol. (2007) 9:654–9. doi: 10.1038/ncb1596
153. Turchinovich A, Burwinkel B. Distinct AGO1 and AGO2 associated miRNA profiles in human cells and blood plasma. RNA Biol. (2012) 9:1066–75. doi: 10.4161/rna.21083
154. Nedaeinia R, Manian M, Jazayeri MH, Ranjbar M, Salehi R, Sharifi M, et al. Circulating exosomes and exosomal microRNAs as biomarkers in gastrointestinal cancer. Cancer Gene Ther. (2017) 24:48–56. doi: 10.1038/cgt.2016.77
155. Zhang J, Li S, Li L, Li M, Guo C, Yao J, et al. Exosome and exosomal microRNA: trafficking, sorting, and function. Genomics Proteomics Bioinf. (2015) 13:17–24. doi: 10.1016/j.gpb.2015.02.001
156. Ali A, Hadlich F, Abbas MW, Iqbal MA, Tesfaye D, Bouma GJ, et al. MicroRNA-mRNA networks in pregnancy complications: A comprehensive downstream analysis of potential biomarkers. Int J Mol Sci. (2021) 22:2313. doi: 10.3390/ijms22052313
157. Ali A, Bouma GJ, Anthony RV, Winger QA. The role of LIN28-let-7-ARID3B pathway in placental development. Int J Mol Sci. (2020) 21:3637. doi: 10.3390/ijms21103637
158. Addo KA, Palakodety N, Hartwell HJ, Tingare A, Fry RC. Placental microRNAs: Responders to environmental chemicals and mediators of pathophysiology of the human placenta. Toxicol Rep. (2020) 7:1046–56. doi: 10.1016/j.toxrep.2020.08.002
159. Kulshreshtha R, Davuluri RV, Calin GA, Ivan M. A microRNA component of the hypoxic response. Cell Death differentiation. (2008) 15:667–71. doi: 10.1038/sj.cdd.4402310
160. Sayed AS, Xia K, Salma U, Yang T, Peng J. Diagnosis, prognosis and therapeutic role of circulating miRNAs in cardiovascular diseases. Heart Lung circulation. (2014) 23:503–10. doi: 10.1016/j.hlc.2014.01.001
161. Bidarimath M, Khalaj K, Kridli RT, Kan FW, Koti M, Tayade C. Extracellular vesicle mediated intercellular communication at the porcine maternal-fetal interface: A new paradigm for conceptus-endometrial cross-talk. Sci Rep. (2017) 7:40476. doi: 10.1038/srep40476
162. Wei Q, Wang Y, Ma K, Li Q, Li B, Hu W, et al. Extracellular vesicles from human umbilical cord mesenchymal stem cells facilitate diabetic wound healing through miR-17-5p-mediated enhancement of angiogenesis. Stem Cell Rev Rep. (2022) 18:1025–40. doi: 10.1007/s12015-021-10176-0
163. Jia L, Zhou X, Huang X, Xu X, Jia Y, Wu Y, et al. Maternal and umbilical cord serum-derived exosomes enhance endothelial cell proliferation and migration. FASEB journal: Off Publ Fed Am Societies Exp Biol. (2018) 32:4534–43. doi: 10.1096/fj.201701337RR
164. Esser JS, Saretzki E, Pankratz F, Engert B, Grundmann S, Bode C, et al. Bone morphogenetic protein 4 regulates microRNAs miR-494 and miR-126-5p in control of endothelial cell function in angiogenesis. Thromb haemostasis. (2017) 117:734–49. doi: 10.1160/th16-08-0643
165. Xiong ZH, Wei J, Lu MQ, Jin MY, Geng HL. Protective effect of human umbilical cord mesenchymal stem cell exosomes on preserving the morphology and angiogenesis of placenta in rats with preeclampsia. Biomedicine pharmacotherapy = Biomedecine pharmacotherapie. (2018) 105:1240–7. doi: 10.1016/j.biopha.2018.06.032
166. Liang H, Ge F, Xu Y, Xiao J, Zhou Z, Liu R, et al. miR-153 inhibits the migration and the tube formation of endothelial cells by blocking the paracrine of angiopoietin 1 in breast cancer cells. Angiogenesis. (2018) 21:849–60. doi: 10.1007/s10456-018-9630-9
167. Sideridou M, Zakopoulou R, Evangelou K, Liontos M, Kotsinas A, Rampakakis E, et al. Cdc6 expression represses E-cadherin transcription and activates adjacent replication origins. J Cell Biol. (2011) 195:1123–40. doi: 10.1083/jcb.201108121
168. Li K, Li R, Zhao Z, Feng C, Liu S, Fu Q. Therapeutic potential of mesenchymal stem cell-derived exosomal miR-296-5p and miR-337-3p in age-related erectile dysfunction via regulating PTEN/PI3K/AKT pathway. Biomedicine pharmacotherapy = Biomedecine pharmacotherapie. (2023) 167:115449. doi: 10.1016/j.biopha.2023.115449
169. Liang H, Xiao J, Zhou Z, Wu J, Ge F, Li Z, et al. Hypoxia induces miR-153 through the IRE1α-XBP1 pathway to fine tune the HIF1α/VEGFA axis in breast cancer angiogenesis. Oncogene. (2018) 37:1961–75. doi: 10.1038/s41388-017-0089-8
170. Choi SY, Yun J, Lee OJ, Han HS, Yeo MK, Lee MA, et al. MicroRNA expression profiles in placenta with severe preeclampsia using a PNA-based microarray. Placenta. (2013) 34:799–804. doi: 10.1016/j.placenta.2013.06.006
171. Zhao G, Yang C, Yang J, Liu P, Jiang K, Shaukat A, et al. Placental exosome-mediated Bta-miR-499-Lin28B/let-7 axis regulates inflammatory bias during early pregnancy. Cell Death disease. (2018) 9:704. doi: 10.1038/s41419-018-0713-8
172. Takahashi H, Ohkuchi A, Kuwata T, Usui R, Baba Y, Suzuki H, et al. Endogenous and exogenous miR-520c-3p modulates CD44-mediated extravillous trophoblast invasion. Placenta. (2017) 50:25–31. doi: 10.1016/j.placenta.2016.12.016
173. Cocco E, Paladini F, Macino G, Fulci V, Fiorillo MT, Sorrentino R. The expression of vasoactive intestinal peptide receptor 1 is negatively modulated by microRNA 525-5p. PloS One. (2010) 5:e12067. doi: 10.1371/journal.pone.0012067
174. Zhang R, Zhao J, Xu J, Wang J, Jia J. miR-526b-3p functions as a tumor suppressor in colon cancer by regulating HIF-1α. Am J Trans Res. (2016) 8:2783–9.
175. Kambe S, Yoshitake H, Yuge K, Ishida Y, Ali MM, Takizawa T, et al. Human exosomal placenta-associated miR-517a-3p modulates the expression of PRKG1 mRNA in Jurkat cells. Biol reproduction. (2014) 91:129. doi: 10.1095/biolreprod.114.121616
176. Chiarello DI, Salsoso R, Toledo F, Mate A, Vázquez CM, Sobrevia L. Foetoplacental communication via extracellular vesicles in normal pregnancy and preeclampsia. Mol aspects Med. (2018) 60:69–80. doi: 10.1016/j.mam.2017.12.002
177. Pillay P, Moodley K, Moodley J, Mackraj I. Placenta-derived exosomes: potential biomarkers of preeclampsia. Int J nanomedicine. (2017) 12:8009–23. doi: 10.2147/ijn.S142732
178. Yoffe L, Polsky A, Gilam A, Raff C, Mecacci F, Ognibene A, et al. Early diagnosis of gestational diabetes mellitus using circulating microRNAs. Eur J endocrinology. (2019) 181:565–77. doi: 10.1530/eje-19-0206
179. Hromadnikova I, Kotlabova K, Ondrackova M, Kestlerova A, Novotna V, Hympanova L, et al. Circulating C19MC microRNAs in preeclampsia, gestational hypertension, and fetal growth restriction. Mediators inflammation. (2013) 2013:186041. doi: 10.1155/2013/186041
180. Khalyfa A, Kheirandish-Gozal L, Bhattacharjee R, Khalyfa AA, Gozal D. Circulating microRNAs as potential biomarkers of endothelial dysfunction in obese children. Chest. (2016) 149:786–800. doi: 10.1378/chest.15-0799
181. Ma R, Liang Z, Shi X, Xu L, Li X, Wu J, et al. Exosomal miR-486-5p derived from human placental microvascular endothelial cells regulates proliferation and invasion of trophoblasts via targeting IGF1. Hum Cell. (2021) 34:1310–23. doi: 10.1007/s13577-021-00543-x
182. Truong G, Guanzon D, Kinhal V, Elfeky O, Lai A, Longo S, et al. Oxygen tension regulates the miRNA profile and bioactivity of exosomes released from extravillous trophoblast cells - Liquid biopsies for monitoring complications of pregnancy. PloS One. (2017) 12:e0174514. doi: 10.1371/journal.pone.0174514
183. Cooper JC. The effect of placental syncytiotrophoblast microvillous membranes from normal and pre-eclamptic women on the growth of endothelial cells. vitro. Br J obstetrics gynaecology. (1994) 101:559. doi: 10.1111/j.1471-0528.1994.tb13171.x
184. Knight M, Redman CW, Linton EA, Sargent IL. Shedding of syncytiotrophoblast microvilli into the maternal circulation in pre-eclamptic pregnancies. Br J obstetrics gynaecology. (1998) 105:632–40. doi: 10.1111/j.1471-0528.1998.tb10178.x
185. Bavishi C, Messerli FH, Kadosh B, Ruilope LM, Kario K. Role of neprilysin inhibitor combinations in hypertension: insights from hypertension and heart failure trials. Eur Heart J. (2015) 36:1967–73. doi: 10.1093/eurheartj/ehv142
186. Gill M, Motta-Mejia C, Kandzija N, Cooke W, Zhang W, Cerdeira AS, et al. Placental syncytiotrophoblast-derived extracellular vesicles carry active NEP (Neprilysin) and are increased in preeclampsia. Hypertension (Dallas Tex: 1979). (2019) 73:1112–9. doi: 10.1161/hypertensionaha.119.12707
187. Tannetta D, Collett G, Vatish M, Redman C, Sargent I. Syncytiotrophoblast extracellular vesicles - Circulating biopsies reflecting placental health. Placenta. (2017) 52:134–8. doi: 10.1016/j.placenta.2016.11.008
188. Gardiner C, Tannetta DS, Simms CA, Harrison P, Redman CW, Sargent IL. Syncytiotrophoblast microvesicles released from pre-eclampsia placentae exhibit increased tissue factor activity. PloS One. (2011) 6:e26313. doi: 10.1371/journal.pone.0026313
189. Pillay P, Maharaj N, Moodley J, Mackraj I. Placental exosomes and pre-eclampsia: Maternal circulating levels in normal pregnancies and, early and late onset pre-eclamptic pregnancies. Placenta. (2016) 46:18–25. doi: 10.1016/j.placenta.2016.08.078
190. Ermini L, Ausman J, Melland-Smith M, Yeganeh B, Rolfo A, Litvack ML, et al. A single sphingomyelin species promotes exosomal release of endoglin into the maternal circulation in preeclampsia. Sci Rep. (2017) 7:12172. doi: 10.1038/s41598-017-12491-4
191. Tong M, Chen Q, James JL, Stone PR, Chamley LW. Micro- and nano-vesicles from first trimester human placentae carry flt-1 and levels are increased in severe preeclampsia. Front endocrinology. (2017) 8:174. doi: 10.3389/fendo.2017.00174
192. Javadi M, Rad JS, Farashah MSG, Roshangar L. An insight on the role of altered function and expression of exosomes and microRNAs in female reproductive diseases. Reprod Sci. (2022) 29:1395–407. doi: 10.1007/s43032-021-00556-9
193. Wang W, Sung N, Gilman-Sachs A, Kwak-Kim J. T helper (Th) cell profiles in pregnancy and recurrent pregnancy losses: th1/th2/th9/th17/th22/tfh cells. Front Immunol. (2020) 11:2025. doi: 10.3389/fimmu.2020.02025
194. Collier AY, Smith LA, Karumanchi SA. Review of the immune mechanisms of preeclampsia and the potential of immune modulating therapy. Hum Immunol. (2021) 82:362–70. doi: 10.1016/j.humimm.2021.01.004
195. Wang Y, Du X, Wang J. Transfer of miR-15a-5p by placental exosomes promotes pre-eclampsia progression by regulating PI3K/AKT signaling pathway via CDK1. Mol Immunol. (2020) 128:277–86. doi: 10.1016/j.molimm.2020.10.019
196. Chi Z, Gao Q, Sun Y, Zhou F, Wang H, Shu X, et al. LINC00473 downregulation facilitates trophoblast cell migration and invasion via the miR-15a-5p/LITAF axis in pre-eclampsia. Environ toxicology. (2021) 36:1618–27. doi: 10.1002/tox.23157
197. Poniedziałek-Czajkowska E, Mierzyński R. Could vitamin D be effective in prevention of preeclampsia? Nutrients. (2021) 13:3854. doi: 10.3390/nu13113854
Keywords: biomarkers, epigenomics, exosomes, microRNAs, preeclampsia
Citation: Shan Y, Hou B, Wang J, Chen A and Liu S (2024) Exploring the role of exosomal MicroRNAs as potential biomarkers in preeclampsia. Front. Immunol. 15:1385950. doi: 10.3389/fimmu.2024.1385950
Received: 14 February 2024; Accepted: 08 March 2024;
Published: 19 March 2024.
Edited by:
Payam Behzadi, Islamic Azad University, IranReviewed by:
Abdelazeem Algammal, Suez Canal University, EgyptChiara Agostinis, Institute for Maternal and Child Health Burlo Garofolo (IRCCS), Italy
Copyright © 2024 Shan, Hou, Wang, Chen and Liu. This is an open-access article distributed under the terms of the Creative Commons Attribution License (CC BY). The use, distribution or reproduction in other forums is permitted, provided the original author(s) and the copyright owner(s) are credited and that the original publication in this journal is cited, in accordance with accepted academic practice. No use, distribution or reproduction is permitted which does not comply with these terms.
*Correspondence: Aiping Chen, chenaiping@qdu.edu.cn; Shiguo Liu, liushiguo@qdu.edu.cn