- 1State Key Laboratory of Biotherapy and Cancer Center, West China Hospital, Sichuan University and Collaborative Innovation Center for Biotherapy, Chengdu, China
- 2Department of Hematology and Hematology Research Laboratory, West China Hospital, Sichuan University, Chengdu, China
Extracellular vesicles (EVs) are nanosized lipid particles released by virtually every living cell. EVs carry bioactive molecules, shuttle from cells to cells and transduce signals, regulating cell growth and metabolism. Pathogenic bacteria can cause serious infections via a wide range of strategies, and host immune systems also develop extremely complex adaptations to counteract bacterial infections. As notable carriers, EVs take part in the interaction between the host and bacteria in several approaches. For host cells, several strategies have been developed to resist bacteria via EVs, including expelling damaged membranes and bacteria, neutralizing toxins, triggering innate immune responses and provoking adaptive immune responses in nearly the whole body. For bacteria, EVs function as vehicles to deliver toxins and contribute to immune escape. Due to their crucial functions, EVs have great application potential in vaccines, diagnosis and treatments. In the present review, we highlight the most recent advances, application potential and remaining challenges in understanding EVs in the interaction between the host and bacteria.
1 Introduction
Bacteria are single-celled prokaryotes in which DNA forms circles and is not contained in a defined nucleus. Bacteria are widely distributed around us, and we are interdependent with good (symbiotic) bacteria and fighting against bad (pathogenic) bacteria. Pathogenic bacterial infection is a global threat, and considerable efforts have been made over the years to overcome the diseases. Therefore, with the deepening of research, it has been found that pathogens can change host immune status to avoid immune clearance through various strategies, such as releasing bacterial extracellular vesicles (EVs) (1).
EVs are small compartments surrounded by a lipid bilayer and are unable to replicate (2). “EVs” is a collective term that covers various subtypes, such as exosomes and microvesicles, and both eukaryotic cells and bacteria are able to release EVs (3, 4). In this review, we refer to “hEVs” as EVs from host cells and “bEVs” as bacterial EVs. Resulting from the special generation procedure and structure, EVs contain various bioactive molecules both in the lumen and the surface. Recently, studies have delineated the crucial roles of EVs in biological processes, including influencing metabolism, maintaining homeostasis, and regulating the immune response (4, 5). Observations from several studies have shown that hEVs protect host cells during infection in several ways, such as neutralizing toxins, promoting the release of cytokines and activating adaptive immunity (6, 7). Meanwhile, bacteria release EVs during infection to deliver virulence (8) and escape immune killing (9). However, in some circumstances, hEVs containing bacterial toxins promote disease progression, while bEVs from Lactobacillus reuteri may protect the host from lipopolysaccharide-induced inflammatory responses (10, 11). Thus, how EVs affect the interaction between the host and bacteria has not been fully elucidated.
In this review, we retrieved literature focusing on EVs derived from both host cells and bacteria during infection. We aim to provide an update on recent evidence highlighting new aspects of the role of EVs during infection and discuss the application potential and the challenges facing EV studies.
2 Overview of Extracellular Vesicles
2.1 EVs From Eukaryotic Cells
Eukaryotic cells constantly and naturally release extracellular vesicles in numerous biological processes. From physiological processes, such as regulating metabolism (12), to pathological processes, such as infection (7) and cancer (13), EVs all play crucial roles. EVs are highly heterogeneous and generated in different pathways. Currently, the generation of the three types of EVs is relatively clear, and they are apoptotic bodies, exosomes and microvesicles. Apoptotic bodies are released from the cell plasma membrane by increased hydrostatic pressure after apoptosis (14). Exosomes form through several processes: (a) invagination of the endosomal membrane; (b) formation of multivesicular bodies; (c) fusion of multivesicular bodies with the plasma membrane; and (d) release from the cell (15). Microvesicles form by a direct outward budding from the cell membrane. Consistent with their generation procedure, EVs are filled with cytosolic proteins such as HSC70, Alix and TSG101 (16). EVs also contain transmembrane proteins such as CD9, CD63 and CD81 (17, 18). Other molecules in the cell matrix, such as RNA, may be encapsulated into EVs during generation, which enables EVs to transfer regulatory messages between cells (19).
2.2 EVs From Bacteria
Bacterial EVs (bEVs) were first reported in Gram-negative bacteria in the 1960s (20), while bEVs from Gram-positive bacteria were not reported until 1990 (21). Due to the existence of the thick peptidoglycan cell wall and the lack of periplasmic space and outer membrane in the cell wall, it was thought to be difficult for Gram-positive bacteria to release bEVs; thus, early studies mainly focused on bEVs from Gram-negative bacteria (22, 23). Outer membrane vesicles (OMVs) are well-explored bEVs from Gram-negative bacteria. OMVs bud from the outer layer of the Gram-negative bacterial cell wall and consist of lipopolysaccharide (LPS) and outer-membrane proteins, which are consistent with their cell walls (3). Apart from OMVs, outer-inner membrane vesicles (O-IMVs) are also found in Gram-negative bacteria (24). This kind of bEV contains both outer membranes and cytoplasmic membranes (25) and may be generated by explosive cell lysis (26). Furthermore, bEVs are also detected in Gram-positive bacteria despite their thick cell walls (27). Little is known about the mechanism, and they may be generated by turgor pressure from the cell wall (28) or cell wallmodifying enzymes (29). The last kind of bEV is nanotube, which is tube-like protrusions of the bacterial membrane and functions as bridges between bacteria for substance exchange (30). BEVs play important roles in bacterial biological processes, including transferring DNA (31), killing other bacteria (32), neutralizing phages (33) and transferring virulence to host cells (8).
3 Interaction Between Host and Bacteria via EVs
3.1 HEVs in the Interaction Between Host and Bacteria
After infection, host-derived EVs (hEVs) demonstrate both antibacterial and probacterial effects under different conditions (7, 34, 35). On the one hand, the host could resist infection through hEVs in several ways. First, damaged membranes induced by bacterial toxins are repaired by releasing hEVs, which is promoted by the calcium-activated lipid scramblase TMEM16F (36) (Figure 1A). Second, infected cells expel bacteria through hEVs. When infected with uropathogenic Escherichia coli, a lysosomal channel called TRPML3 sensed the change in lysosome pH and led to Ca2+ efflux, and this efflux triggered hEVs release to expel bacteria (37) (Figure 1B). Third, hEVs protect host cells from toxins by binding to toxins as decoys (7). In these hEVs, a special protein, named ADAM10 (38), existed on the surface and was able to bind to alpha-toxin of methicillin-resistant Staphylococcus aureus, which enabled hEVs to neutralize toxin (Figure 1C). Fourth, hEVs participate in innate immunity. Mtb-infected macrophages released hEVs to healthy macrophages, provoking a RIG-I/MAVS/TBK1/IRF3 RNA sensing pathway and the generation of type I interferon (39). HEVs from LPS-activated macrophages induced MyD88/NF-κB signaling and promoted the expression of cytokines and chemokines, such as IL-4, IL-1β, IFN-γ, CCL4 and CCL19 (40) (Figure 1D). Fifth, hEVs participate in adaptive immunity by contributing to the T-cell response. Phagocytic events enhanced DCs to release hEVs, and these hEVs demonstrated MHC molecules on the surface, enabling hEV antigen-presenting capacity (6). Infected macrophages were also able to release MHC molecule-demonstrating hEVs for adaptive responses (41) (Figure 1E).
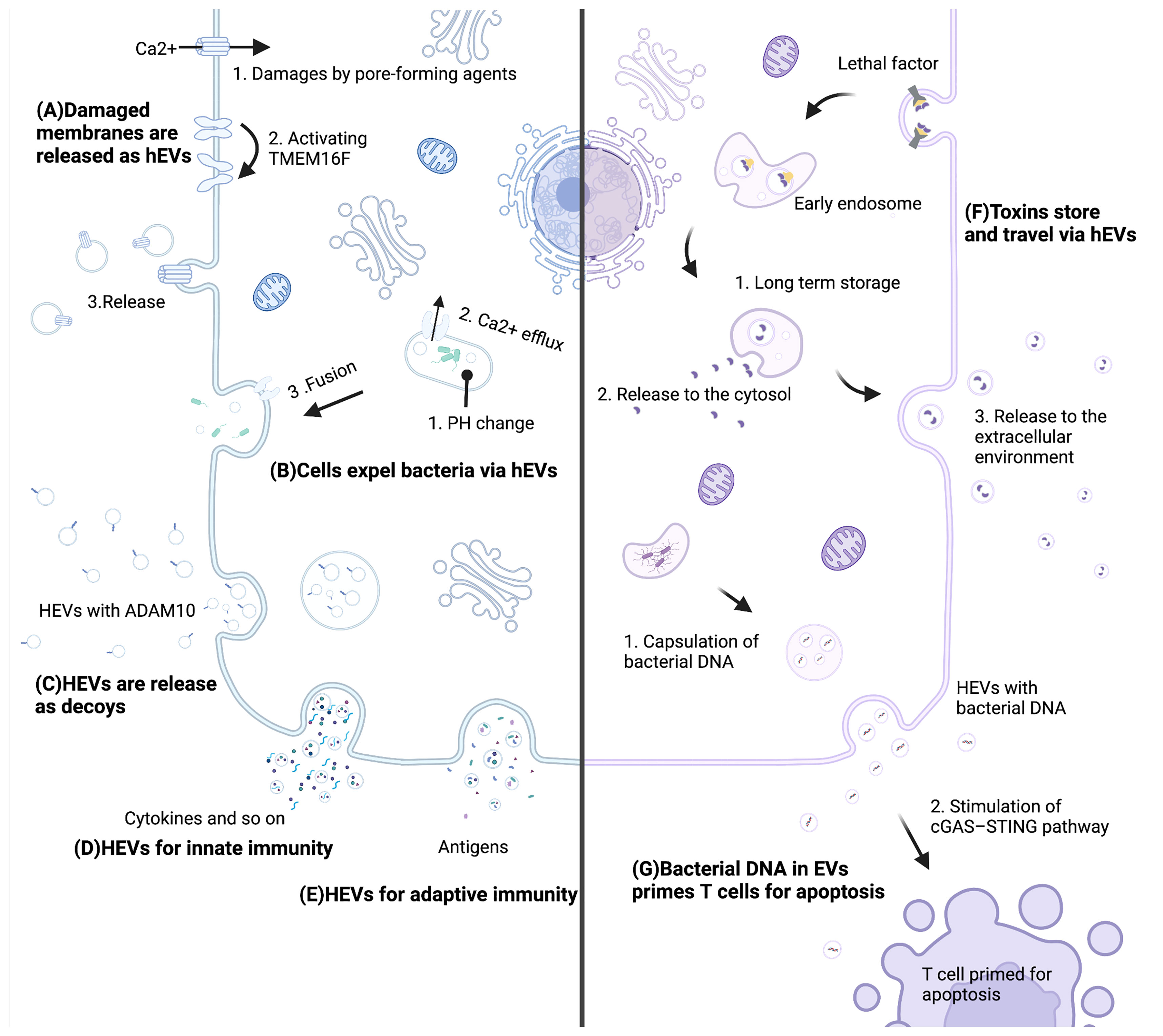
Figure 1 Mechanisms of hEVs in the interaction between the host and bacteria. (A) Pore-forming agents lead to Ca2+ influx and activate TMEM16F. Subsequently, damaged membranes are repaired by releasing hEVs. (B) A pH change leads to Ca2+ efflux, and this efflux triggers hEVs release to expel bacteria. (C) Host cells release ADAM10-containing hEVs to neutralize toxins. (D) Cells release molecules such as cytokines via hEVs for innate immunity. (E) Cells present antigens via hEVs for adaptive immunity. (F) Lethal factors of anthrax store in late endosomes sheltering from degradation and are released to the cytosol and the extracellular environment. (G) Bacterial DNA of Listeria monocytogenes is encapsulated into hEVs, and these hEVs stimulate the cGAS-STING pathway of T cells, which primes T cells for apoptosis. This figure was created with BioRender.com.
On the other hand, pathogenic bacteria attack and damage host cells through hEVs. Lethal factor is one of two parts of anthrax lethal toxin. Lethal factors could be translocated to the lumen of host vesicles during assembly and endocytosis, where they were sheltered from degradation for days and could be delivered to the extracellular medium, leading to damage to the host (10) (Figure 1F). Moreover, Listeria monocytogenes took advantage of hEVs to deliver its DNA to bystander cells. These bacterial DNA-containing hEVs inhibited T-cell proliferation and induced apoptosis (42). Thus, Listeria monocytogenes impaired antimicrobial defense via hEVs (Figure 1G). In terms of probiotics, the host can release hEVs to modulate probiotic functions. Colonic epithelial cells released hEVs to Lactobacillus rhamnosus GG, which might mediate the production of p40. Consequently, Lactobacillus rhamnosus GG released p40 into the enteric cavity, protecting epithelial cells against inflammation (35).
3.2 BEVs in the Interaction Between Host and Bacteria
Bacterial EVs possess many functions in the interaction between the host and the bacteria. One of the most important functions is to transfer virulence to the host cells. For example, VacA, a Helicobacter pylori (H. pylori) toxin, was constitutively released via bEVs, accounting for 25% of total VacA (43). This bEV-associated VacA was internalized by gastric epithelial cells, which promoted low-grade gastritis to support the persistence of H. pylori (44). Bacteria exploit bEVs as an alternative secretory pathway to cover the shortage of other secretory systems, and the mechanisms by which bEV-associated toxins enter host cells may be completely different (45). Compared with free toxin, bEVs provide protection against digestion by intestinal proteases, thus allowing the bacteria to target host cells distant from the primary colonization sites (46). BEVs are able to travel through barriers. In an in vitro intestinal epithelial cell model, bEVs could be transported across a polarized cell monolayer with an estimated average uptake efficiency of 30%, suggesting the possibility that bEVs might travel across the gastrointestinal epithelium (47). Moreover, studies showed that bEVs were successfully delivered to the brain crossing the blood–brain barrier and were taken up by meningeal macrophages in mice (48, 49).
Apart from virulence transfer, bEVs contribute to the immune evasion of bacteria. Bacteria release bEVs as strategies to resist antimicrobial molecules from the host. BEVs from Salmonella expressed PagC, which was able to bind to complement component C3b and complement inhibitor factor H. These PagC+ bEVs recruited complement inhibitor factor H and degraded active C3 (50). KatA in H. pylori EVs detoxified reactive oxygen species released by immune cells and rescued bacteria from killing during the respiratory burst (51). BEVs are also released to resist polymyxin (52) and antimicrobial fatty acids (53), protecting bacteria from harm.
4 EVs in Specific Systems and Diseases
Each host system has different strategies and characteristics to address the invasion of pathogenic bacteria. The latest studies have highlighted EV-mediated interactions in several systems, such as the respiratory, gastrointestinal and urinary systems (Table 1).
4.1 Respiratory System
The respiratory system is one of the most investigated systems in terms of EVs. The main topics involve mycobacterial species such as Bacillus Calmette-Guerin (BCG), Mycobacterium tuberculosis (Mtb) and Pseudomonas aeruginosa (P. aeruginosa).
4.1.1 HEVs in the Respiratory System
Studies have analyzed exosomal RNA from Mtb-infected or BCG-infected macrophages in vitro and revealed that Mtb infection led to changes in RNA in hEVs, including transcripts that were involved in the antibacterial response and miRNAs that might be associated with metabolism and energy production (54, 55). Interestingly, mycobacterial transcripts were also found in hEVs derived from macrophages infected in vitro by Mtb (54), and Mtb RNA might be encapsulated through a SecA2-dependent pathway (39). For hEV proteins, 68 proteins originating from hEVs that were released by Mtb-infected macrophages were significantly different compared with the control and were mainly involved in vesicular formation, antigen processing and immune function (56). Forty-one mycobacterial proteins were also identified, and these proteins were highly immunogenic, suggesting a proinflammatory function (57). These changes in the constituents of hEVs usually enhance innate and adaptive immune responses. HEVs from Mtb-infected cells contributed to the release of cytokines and recruitment of host cells (58). HEVs containing mycobacterial antigens were able to activate the adaptive immune response. Subsequently, Mtb-specific CD4+ T cells proliferated (59).
Pseudomonas aeruginosa is a common opportunistic pathogen in the lung. After exposure to P. aeruginosa, airway epithelial cells released hEVs to macrophages, and these stimulated macrophages released more cytokines and increased the expression of innate immune genes, suggesting an EV-mediated mechanism between bacteria-infected cells and immune cells during infection (60).
4.1.2 BEVs in the Respiratory System
Several mycobacterial species, such as the medically important BCG and Mtb, all possess the ability to release membrane vesicles despite their thick cell walls (28). When Mtb infected macrophages, the macrophages released two distinct EVs: one enriched in host cell markers and the other enriched in Mtb molecules. The release of the latter one was dependent on bacterial viability, which implied that Mtb might release bacterial vesicles during the intracellular stay (61). Mice from the group that were exposed to Mtb EVs first and then infected with Mtb showed accelerated local inflammation, and increased bacterial replication was seen in the lungs and spleens, suggesting a pathogenic role of bEVs during tuberculosis (62).
BEVs from P. aeruginosa are complex entities composed of flagellin, lipopolysaccharide and other proteins and are able to induce an inflammatory response in host cells. When mice were exposed to bEVs from P. aeruginosa, neutrophils and macrophages accumulated, and multiple cytokines increased, including CXCL1, CCL2, IL-1β, TNF-α, IL-6, and IFN-γ (63). This innate immune response is elicited in several pathways. Both toll-like receptor (TLR)2 and TLR4 knockout mice showed a slight reduction in inflammatory responses, suggesting the involvement of toll-like receptors in the response (63). Apart from toll-like receptors, peptidoglycan in bEVs is recognized by NOD-like receptors in the cytoplasm (64). Inflammasomes, including NLRP3 and NLRC4, are detected during infection (64), and flagellin in bEVs may be a key ligand for NLRC4 inflammasome activation (65). However, in some diseases, bEVs may benefit the host by regulating regulatory T cells. In asthma, bEVs increased the regulatory T-cell response and decreased the Th2 response, which led to the inhibition of airway hyperresponsiveness, inflammation and serum IgE secretion and offered protection against allergic sensitization (66). In ischemia–reperfusion injury, bEV preconditioning attenuated tissue injury and reduced the total protein concentration. This protective effect was achieved by regulating the balance of regulatory T cells and Th17 cells through the Tim-3 and TLR4/NF-κB pathways (67).
4.2 Gastrointestinal System
In terms of the gastrointestinal system, the focus of EV studies relies on H. pylori and Salmonella typhimurium.
4.2.1 HEVs in the Gastrointestinal System
HEVs released by H. pylori-infected cells in the stomach can reach the blood circulation and directly affect the cardiovascular system. It was reported that in H. pylori-infected patient serum, hEVs contained cagA, a major virulence factor of H. pylori. These hEVs promoted foam cell formation and aggravated atherosclerosis (68, 69). Increased exosomal miR-25 was found in H. pylori-infected patient blood and was associated with negative cardiovascular events (70). Intriguingly, in addition to negative effects on vascular endothelial cells, a study implied that hEVs from H. pylori-infected cells demonstrated protumor effects. H. pylori-infected gastric cancer cells released hEVs that contained mesenchymal‐epithelial transition factor and educated macrophages toward a protumorigenesis phenotype (71).
HEVs from S. Typhimurium-infected macrophages affect innate and adaptive immunity. For innate immunity, one subtype (CD63+ and CD9+) of infected macrophage-derived EVs triggered the secretion of TLR4-dependent tumor necrosis factor alpha and other cytokines, such as RANTES, GM-CSF, and G-CSF (72). Regarding adaptive immunity, another subtype of hEVs was composed of bacterial antigens, and these hEVs promoted T-helper 1-cell activation and anti-S. Typhimurium antibody production (73).
4.2.2 BEVs in the Gastrointestinal System
Quantitative proteomic analyses revealed that bEVs from H. pylori contained more than 400 proteins, including the typical virulence factors of H. pylori, such as vacA and cagA (8). This constitutively shed bEVs play a role in promoting the low-grade gastritis associated during H. pylori infection (44). Toxins in bEVs also suppress T-cell proliferation. This effect is not through a direct effect on T cells but results from the induction of COX-2 expression in monocytes (74). Apart from inflammation, cancer signaling pathway alterations have been identified, including metabolic pathways and the PI3K-Akt signaling pathway (8). VacA in bEVs might contribute to carcinogenesis during H. pylori infection (75). Moreover, CagA and LPS from bEVs might injure the endothelium and promote atherosclerotic plaque formation. These processes might be associated with the activation of the ROS/NF-κB signaling pathway (76).
Salmonella enterica serovar Typhimurium is an intracellular bacterium and is able to survive in macrophages. To promote bacterial survival and spread, strategies have evolved, and releasing bEVs during the intracellular stay is one of these strategies. After infection with Salmonella Typhimurium, epithelial cells released bEV-like vesicles. The release of this vesicle depended on intact Salmonella-containing vacuoles in infected cells. This vesicle damaged bystander cells through several processes: (a) anterograde transport on microtubule and actin tracks and release from infected host cells; (b) internalization by bystander cells through active endocytosis; and (c) retrograde transport through the Golgi complex and causing cell damages (77). New virulence factors, including PagK, PagJ, and STM2585A, were also found in bEVs (78). However, the host also develops mechanisms to defend against these attacks. AMPK in host cells was able to recognize bEVs and subsequently activate the autophagy pathway before bacterial invasion (79).
4.3 Urinary System
4.3.1 HEVs in the Gastrointestinal System
Uropathogenic Escherichia coli (UPEC) is a causative agent in urinary tract infection. During infection, HEVs show both protective and harmful effects. EVs from bladder epithelial cells were enriched in the iron-binding glycoprotein lactoferrin, and this type of hEV reduced bacterial adherence and promoted neutrophil functions (80). However, EVs from urothelial cells in a pyroptotic state led to barrier damage. These hEVs were full of IL-1beta and IL-18, which recruited mast cells. As a consequence, mast cells released tryptase and damaged barrier function via the tryptase-PAR2 axis (81).
4.3.2 BEVs in the Gastrointestinal System
UPEC produces a toxin called cytotoxic necrotizing factor type 1 (CNF1). This toxin constitutively activates small GTPases of the Rho family and results in decreased membrane fluidity in mouse polymorphonuclear leukocytes (84). CNF1 was detected in UPEC OMVs, and in vitro experiments confirmed CNF1 delivery into host cells by OMVs (82). Further study showed that CNF1-containing OMVs were transferred to polymorphonuclear leukocytes, negatively affecting the efficacy of the acute inflammatory response to these organisms (83).
5 Applications
As crucial participators in the interaction between the host and pathogenic bacteria, growing evidence suggests that EVs have great potential in vaccines, diagnosis and treatment (Figure 2).
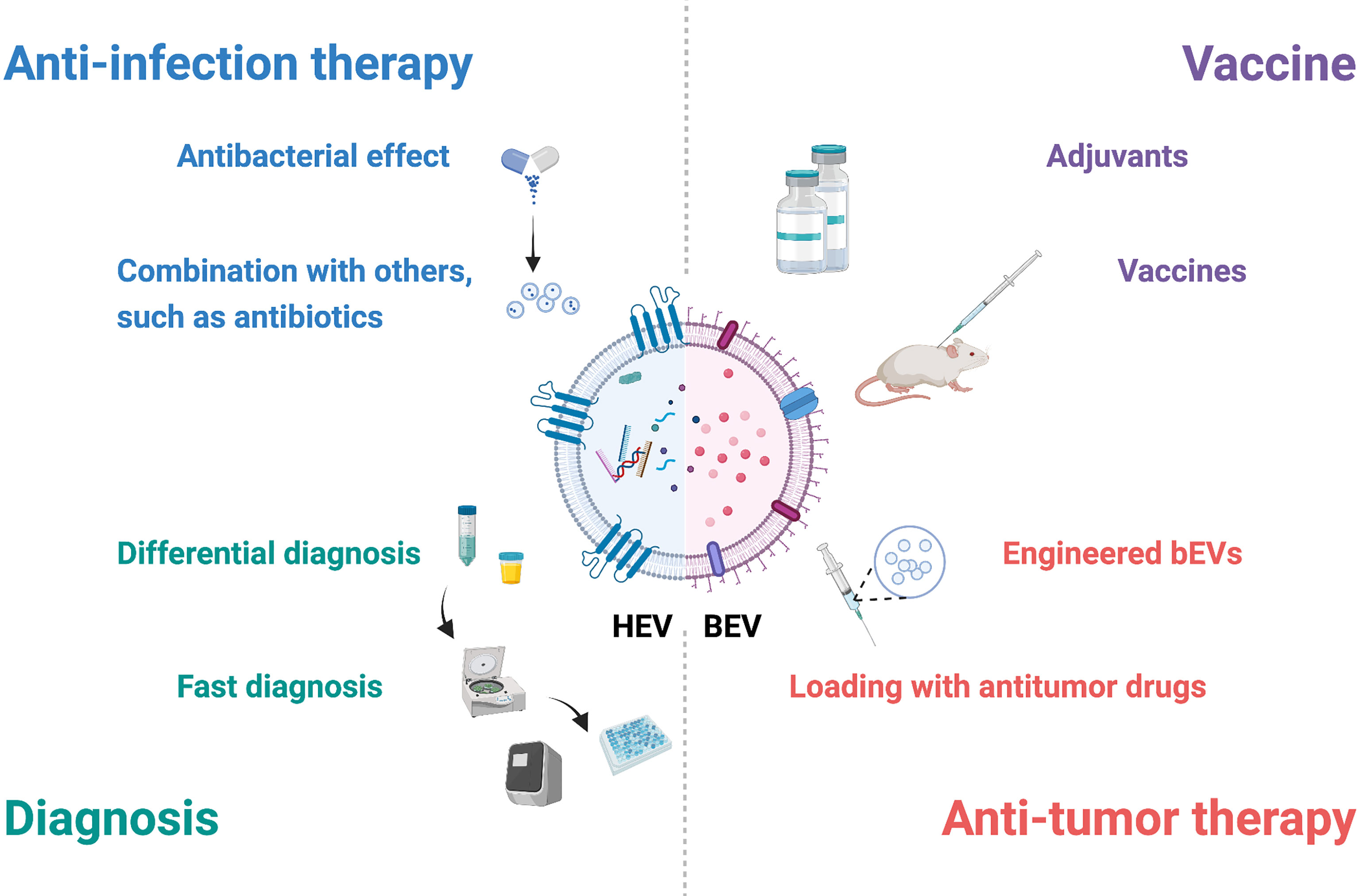
Figure 2 Potential applications of EVs in interactions between the host and bacteria. EVs have potential in diagnosis, anti-infection therapy, vaccines and antitumor therapy. Combined with specific molecules and other techniques, hEVs may be utilized for fast diagnosis and differential diagnosis. HEVs from specific cells, such as mesenchymal stem cells, possess antibacterial effects, and hEVs combined with other materials may show better therapeutic effects. BEVs contain many pathogen-associated molecular patterns that provoke innate and adaptive immune responses and are suitable for adjuvants and vaccines. Engineered bEVs express specific proteins that demonstrate antitumor effects. Meanwhile, these bEVs are able to load antitumor drugs, showing potential in antitumor therapy. This figure was created with BioRender.com.
5.1 HEVs for Applications
HEVs carry specific molecules in both the surface and lumen, and these biomarkers differ according to the sources and diseases, which shows the potential for diagnosis. Recognition of biomarkers in hEVs with a fast examination technique provides better and faster results in diagnosis. For example, the combination of hEV DNA and droplet digital PCR presented higher sensitivity for tuberculosis detection, especially when bacterial loads were low (85). HEVs can also be used in differential diagnosis. The ability to aggregate with bacteria ensured hEVs to distinguish bacterial infection from noninfectious inflammation (86). Differences in akt and CD9 from urinary EVs might be markers for differentiating urinary tract infection from asymptomatic bacteriuria (87).
Specific subtypes of hEVs exert therapeutic effects. MSC-derived EVs protected against infection in acute lung injury (88). Combination with other materials, such as antibiotics and other nanomaterials, also improves the therapeutic effects. Either administrating hEVs with antibiotics or using antibiotic-containing hEVs provided better outcomes in infected mice (89). A dressing with a combination of silver nanoparticles and hEVs promoted angiogenesis and wound healing in P. aeruginosa-infected mice (90).
5.2 BEVs for Applications
BEVs contain many pathogen-associated molecular patterns, such as flagella, peptidoglycans and LPS, which not only provoke the innate immune response but also induce the adaptive immune response. Due to their immunogenicity, bEVs are suitable for adjuvants and vaccines. Compared with the standard adjuvants, bEVs from H. pylori efficiently triggered the Th1 immune response, and the response was skewed toward Th2- and Th17-biased immunity against H. pylori, suggesting a higher efficiency in inducing immune responses (91). BEVs from flagellin-deficient Salmonella typhimurium (92) and Pseudomonas pseudomallei (93) also showed competency as adjuvants. In terms of vaccines, bEVs are able to trigger both humoral immunity (94) and cellular immunity (95) and generate memory cells (96). A retrospective case–control study demonstrated that the outer membrane vesicle meningococcal B vaccine protected individuals from gonorrhea owing to cross-protection with 31% effectiveness (97).
Engineered bEVs are suitable vehicles in antitumor treatment. Several ways have been developed to engineer bacteria: (1) expressing tumor-targeting ligands in bEVs to specifically bind to tumor tissues and to increase drug delivery efficiency (98); (2) expressing tumor proteins to induce antitumor responses as vaccines (99); and (3) expressing proteins such as PD1 to enhance the immune response (100). Meanwhile, as natural cargos, bEVs possess the ability to deliver drugs. Chemotherapeutic drugs, such as doxorubicin (101), and gene therapy drugs, such as siRNA (98), can be packaged into bEVs. With these two advantages, bEVs have great potential in antitumor therapy.
6 Conclusion and Perspective
Recent advances in EV research have greatly enhanced our understanding of how the host interacts with pathogenic bacteria. The explorations of mechanisms in either hosts against bacteria or vice versa shed light on the interaction between host and bacteria from a different perspective. EVs participate in infection and anti-infection activities in almost every system, varying from the respiratory system to the urinary system. It is both intriguing and challenging to unravel the complex mechanisms underlying EV regulation and their significance for infectious diseases.
However, inadequacies still exist, and one of the most problematic issues is ignorance of heterogeneity. Heterogeneity is prominent in EVs involving size (102) and components (103), and several technologies have been used to tackle this difficult problem from different aspects (18, 104, 105). EVs from either host cells or bacteria during infection show great heterogeneity. Differences in purification protocols and EV compositions both contribute to heterogeneity. A study (106) demonstrated that different centrifuge speeds affected EVs. In the 100k pellet, EVs contained Legionella LPS. However, in the 16k pellet, EVs were fewer and represented microparticles. Moreover, even when purified from the same purification protocols, EVs may vary due to their compositions. MiRNA-rich EVs were found in P. aeruginosa pneumonia. These EVs accounted for only 6% of total EVs but contained 39% total RNA. They were actively delivered into immune cells and promoted proinflammatory responses (107). Thus, for future studies, how to recognize and separate the specific subtype may be a main concern. Detailed records of the infection procedure, cell culture conditions and purification protocols and further validation of specific markers in EVs may all decrease the negative influence caused by heterogeneity. In addition to heterogeneity, other topics also draw attention: EV differences in acute and chronic infection, EV storage conditions, large-scale production for clinical applications and so on.
In conclusion, EVs play important roles in the interaction between host and bacteria in various kinds of diseases, and more studies are needed for better understanding and practical application.
Author Contributions
CZ and YZ collected and wrote the manuscript. HL designed the figures and edited the manuscript. YW was responsible for the arrangement of new documents and the language revision. XZ provided guidance and revised this manuscript. All authors contributed to the article and approved the submitted version.
Funding
This work was supported by the National Natural Science Foundation of China (no. 81922042 and 82172285), National Clinical Research Center for Geriatrics, West China Hospital, Sichuan University (no. Z2018B02), Excellent Young Scientist Foundation of Sichuan University (no. 2017SCU04A16), Innovative Spark Foundation of Sichuan University (no. 2018SCUH0032), National Major Scientific and Technological Special Project for “Significant New Drugs Development” (no. 2018ZX09201018-013) and 1·3·5 project of excellent development of discipline of West China Hospital of Sichuan University (No. ZYYC21001).
Conflict of Interest
The authors declare that the research was conducted in the absence of any commercial or financial relationships that could be construed as a potential conflict of interest.
Publisher’s Note
All claims expressed in this article are solely those of the authors and do not necessarily represent those of their affiliated organizations, or those of the publisher, the editors and the reviewers. Any product that may be evaluated in this article, or claim that may be made by its manufacturer, is not guaranteed or endorsed by the publisher.
Abbreviations
EVs, extracellular vesicles; hEVs, extracellular vesicles from host cells; bEVs, extracellular vesicles from bacteria; OMVs, outer membrane vesicles; LPS, lipopolysaccharide; O-IMVs, outer-inner membrane vesicles; H. pylori, Helicobacter pylori; BCG, Bacillus Calmette-Guerin; Mtb, Mycobacterium tuberculosis; P. aeruginosa, Pseudomonas aeruginosa; TLR, toll-like receptor; UPEC, uropathogenic Escherichia coli; CNF1, cytotoxic necrotizing factor type 1.
References
1. Kaparakis-Liaskos M, Ferrero RL. Immune Modulation by Bacterial Outer Membrane Vesicles. Nat Rev Immunol (2015) 15(6):375–87. doi: 10.1038/nri3837
2. Théry C, Witwer KW, Aikawa E, Alcaraz MJ, Anderson JD, Andriantsitohaina R, et al. Minimal Information for Studies of Extracellular Vesicles 2018 (MISEV2018): A Position Statement of the International Society for Extracellular Vesicles and Update of the MISEV2014 Guidelines. J Extracell Vesicles (2018) 7(1):1535750. doi: 10.1080/20013078.2018.1535750
3. Toyofuku M, Nomura N, Eberl L. Types and Origins of Bacterial Membrane Vesicles. Nat Rev Microbiol (2019) 17(1):13–24. doi: 10.1038/s41579-018-0112-2
4. Yáñez-Mó M, Siljander PR, Andreu Z, Zavec AB, Borràs FE, Buzas EI, et al. Biological Properties of Extracellular Vesicles and Their Physiological Functions. J Extracell Vesicles (2015) 4:27066. doi: 10.3402/jev.v4.27066
5. Wang J, Yao Y, Chen X, Wu J, Gu T, Tang X. Host Derived Exosomes-Pathogens Interactions: Potential Functions of Exosomes in Pathogen Infection. BioMed Pharmacother (2018) 108:1451–9. doi: 10.1016/j.biopha.2018.09.174
6. Lindenbergh MFS, Wubbolts R, Borg EGF, van 't Veld EM, Boes M, Stoorvogel W. Dendritic Cells Release Exosomes Together With Phagocytosed Pathogen; Potential Implications for the Role of Exosomes in Antigen Presentation. J Extracell Vesicles (2020) 9(1):1798606. doi: 10.1080/20013078.2020.1798606
7. Keller MD, Ching KL, Liang FX, Dhabaria A, Tam K, Ueberheide BM, et al. Decoy Exosomes Provide Protection Against Bacterial Toxins. Nature (2020) 579(7798):260–4. doi: 10.1038/s41586-020-2066-6
8. Wei S, Li X, Wang J, Wang Y, Zhang C, Dai S, et al. Outer Membrane Vesicles Secreted by Helicobacter Pylori Transmitting Gastric Pathogenic Virulence Factors. ACS Omega (2022) 7(1):240–58. doi: 10.1021/acsomega.1c04549
9. Tiku V, Tan MW. Host Immunity and Cellular Responses to Bacterial Outer Membrane Vesicles. Trends Immunol (2021) 42(11):1024–36. doi: 10.1016/j.it.2021.09.006
10. Abrami L, Brandi L, Moayeri M, Brown MJ, Krantz BA, Leppla SH, et al. Hijacking Multivesicular Bodies Enables Long-Term and Exosome-Mediated Long-Distance Action of Anthrax Toxin. Cell Rep (2013) 5(4):986–96. doi: 10.1016/j.celrep.2013.10.019
11. Hu R, Lin H, Wang M, Zhao Y, Liu H, Min Y, et al. Lactobacillus Reuteri-Derived Extracellular Vesicles Maintain Intestinal Immune Homeostasis Against Lipopolysaccharide-Induced Inflammatory Responses in Broilers. J Anim Sci Biotechnol (2021) 12(1):25. doi: 10.1186/s40104-020-00532-4
12. Isaac R, Reis FCG, Ying W, Olefsky JM. Exosomes as Mediators of Intercellular Crosstalk in Metabolism. Cell Metab (2021) 33(9):1744–62. doi: 10.1016/j.cmet.2021.08.006
13. Marar C, Starich B, Wirtz D. Extracellular Vesicles in Immunomodulation and Tumor Progression. Nat Immunol (2021) 22(5):560–70. doi: 10.1038/s41590-021-00899-0
14. Doyle LM, Wang MZ. Overview of Extracellular Vesicles, Their Origin, Composition, Purpose, and Methods for Exosome Isolation and Analysis. Cells (2019) 8(7):727. doi: 10.3390/cells8070727
15. Kalluri R, LeBleu VS. The Biology, Function, and Biomedical Applications of Exosomes. Science (2020) 367(6478):eaau6977. doi: 10.1126/science.aau6977
16. Colombo M, Raposo G, Théry C. Biogenesis, Secretion, and Intercellular Interactions of Exosomes and Other Extracellular Vesicles. Annu Rev Cell Dev Biol (2014) 30:255–89. doi: 10.1146/annurev-cellbio-101512-122326
17. Lai RC, Tan SS, Yeo RW, Choo AB, Reiner AT, Su Y, et al. MSC Secretes at Least 3 EV Types Each With a Unique Permutation of Membrane Lipid, Protein and RNA. J Extracell Vesicles (2016) 5:29828. doi: 10.3402/jev.v5.29828
18. Kowal J, Arras G, Colombo M, Jouve M, Morath JP, Primdal-Bengtson B, et al. Proteomic Comparison Defines Novel Markers to Characterize Heterogeneous Populations of Extracellular Vesicle Subtypes. Proc Natl Acad Sci U.S.A. (2016) 113(8):E968–77. doi: 10.1073/pnas.1521230113
19. O'Brien K, Breyne K, Ughetto S, Laurent LC, Breakefield XO. RNA Delivery by Extracellular Vesicles in Mammalian Cells and Its Applications. Nat Rev Mol Cell Biol (2020) 21(10):585–606. doi: 10.1038/s41580-020-0251-y
20. Bishop DG, Work E. An Extracellular Glycolipid Produced by Escherichia Coli Grown Under Lysine-Limiting Conditions. Biochem J (1965) 96(2):567–76. doi: 10.1042/bj0960567
21. Dorward DW, Garon CF. DNA Is Packaged Within Membrane-Derived Vesicles of Gram-Negative But Not Gram-Positive Bacteria. Appl Environ Microbiol (1990) 56(6):1960–2. doi: 10.1128/aem.56.6.1960-1962.1990
22. Schwechheimer C, Kuehn MJ. Outer-Membrane Vesicles From Gram-Negative Bacteria: Biogenesis and Functions. Nat Rev Microbiol (2015) 13(10):605–19. doi: 10.1038/nrmicro3525
23. Kulp A, Kuehn MJ. Biological Functions and Biogenesis of Secreted Bacterial Outer Membrane Vesicles. Annu Rev Microbiol (2010) 64:163–84. doi: 10.1146/annurev.micro.091208.073413
24. Pérez-Cruz C, Delgado L, López-Iglesias C, Mercade E. Outer-Inner Membrane Vesicles Naturally Secreted by Gram-Negative Pathogenic Bacteria. PloS One (2015) 10(1):e0116896. doi: 10.1371/journal.pone.0116896
25. Pérez-Cruz C, Carrión O, Delgado L, Martinez G, López-Iglesias C, Mercade E. New Type of Outer Membrane Vesicle Produced by the Gram-Negative Bacterium Shewanella Vesiculosa M7T: Implications for DNA Content. Appl Environ Microbiol (2013) 79(6):1874–81. doi: 10.1128/aem.03657-12
26. Devos S, Van Putte W, Vitse J, Van Driessche G, Stremersch S, Van Den Broek W, et al. Membrane Vesicle Secretion and Prophage Induction in Multidrug-Resistant Stenotrophomonas Maltophilia in Response to Ciprofloxacin Stress. Environ Microbiol (2017) 19(10):3930–7. doi: 10.1111/1462-2920.13793
27. Gill S, Catchpole R, Forterre P. Extracellular Membrane Vesicles in the Three Domains of Life and Beyond. FEMS Microbiol Rev (2019) 43(3):273–303. doi: 10.1093/femsre/fuy042
28. Brown L, Wolf JM, Prados-Rosales R, Casadevall A. Through the Wall: Extracellular Vesicles in Gram-Positive Bacteria, Mycobacteria and Fungi. Nat Rev Microbiol (2015) 13(10):620–30. doi: 10.1038/nrmicro3480
29. Wang X, Thompson CD, Weidenmaier C, Lee JC. Release of Staphylococcus Aureus Extracellular Vesicles and Their Application as a Vaccine Platform. Nat Commun (2018) 9(1):1379. doi: 10.1038/s41467-018-03847-z
30. Baidya AK, Bhattacharya S, Dubey GP, Mamou G, Ben-Yehuda S. Bacterial Nanotubes: A Conduit for Intercellular Molecular Trade. Curr Opin Microbiol (2018) 42:1–6. doi: 10.1016/j.mib.2017.08.006
31. Fulsundar S, Harms K, Flaten GE, Johnsen PJ, Chopade BA, Nielsen KM. Gene Transfer Potential of Outer Membrane Vesicles of Acinetobacter Baylyi and Effects of Stress on Vesiculation. Appl Environ Microbiol (2014) 80(11):3469–83. doi: 10.1128/aem.04248-13
32. Tashiro Y, Hasegawa Y, Shintani M, Takaki K, Ohkuma M, Kimbara K, et al. Interaction of Bacterial Membrane Vesicles With Specific Species and Their Potential for Delivery to Target Cells. Front Microbiol (2017) 8:571. doi: 10.3389/fmicb.2017.00571
33. Tzipilevich E, Habusha M, Ben-Yehuda S. Acquisition of Phage Sensitivity by Bacteria Through Exchange of Phage Receptors. Cell (2017) 168(1-2):186–99.e12. doi: 10.1016/j.cell.2016.12.003
34. White JR, Dauros-Singorenko P, Hong J, Vanholsbeeck F, Phillips A, Swift S. The Complex, Bidirectional Role of Extracellular Vesicles in Infection. Biochem Soc Trans (2021) 49(2):881–91. doi: 10.1042/bst20200788
35. Yang L, Higginbotham JN, Liu L, Zhao G, Acra SA, Peek RM Jr., et al. Production of a Functional Factor, P40, by Lactobacillus Rhamnosus GG Is Promoted by Intestinal Epithelial Cell-Secreted Extracellular Vesicles. Infect Immun (2019) 87(7):e00113–19. doi: 10.1128/iai.00113-19
36. Wu N, Cernysiov V, Davidson D, Song H, Tang J, Luo S, et al. Critical Role of Lipid Scramblase TMEM16F in Phosphatidylserine Exposure and Repair of Plasma Membrane After Pore Formation. Cell Rep (2020) 30(4):1129–40.e5. doi: 10.1016/j.celrep.2019.12.066
37. Miao Y, Li G, Zhang X, Xu H, Abraham SN. A TRP Channel Senses Lysosome Neutralization by Pathogens to Trigger Their Expulsion. Cell (2015) 161(6):1306–19. doi: 10.1016/j.cell.2015.05.009
38. Panizza E, Cerione RA, Antonyak MA. Exosomes as Sentinels Against Bacterial Pathogens. Dev Cell (2020) 53(2):138–9. doi: 10.1016/j.devcel.2020.03.022
39. Cheng Y, Schorey JS. Extracellular Vesicles Deliver Mycobacterium RNA to Promote Host Immunity and Bacterial Killing. EMBO Rep (2019) 20(3):e46613. doi: 10.15252/embr.201846613
40. Hong Y, Lee J, Vu TH, Lee S, Lillehoj HS, Hong YH. Exosomes of Lipopolysaccharide-Stimulated Chicken Macrophages Modulate Immune Response Through the MyD88/NF-κb Signaling Pathway. Dev Comp Immunol (2020) 115:103908. doi: 10.1016/j.dci.2020.103908
41. Ramachandra L, Qu Y, Wang Y, Lewis CJ, Cobb BA, Takatsu K, et al. Mycobacterium Tuberculosis Synergizes With ATP to Induce Release of Microvesicles and Exosomes Containing Major Histocompatibility Complex Class II Molecules Capable of Antigen Presentation. Infect Immun (2010) 78(12):5116–25. doi: 10.1128/iai.01089-09
42. Nandakumar R, Tschismarov R, Meissner F, Prabakaran T, Krissanaprasit A, Farahani E, et al. Intracellular Bacteria Engage a STING-TBK1-MVB12b Pathway to Enable Paracrine cGAS-STING Signalling. Nat Microbiol (2019) 4(4):701–13. doi: 10.1038/s41564-019-0367-z
43. Ricci V, Chiozzi V, Necchi V, Oldani A, Romano M, Solcia E, et al. Free-Soluble and Outer Membrane Vesicle-Associated VacA From Helicobacter Pylori: Two Forms of Release, A Different Activity. Biochem Biophys Res Commun (2005) 337(1):173–8. doi: 10.1016/j.bbrc.2005.09.035
44. Ismail S, Hampton MB, Keenan JI. Helicobacter Pylori Outer Membrane Vesicles Modulate Proliferation and Interleukin-8 Production by Gastric Epithelial Cells. Infect Immun (2003) 71(10):5670–5. doi: 10.1128/iai.71.10.5670-5675.2003
45. Nice JB, Balashova NV, Kachlany SC, Koufos E, Krueger E, Lally ET, et al. Aggregatibacter Actinomycetemcomitans Leukotoxin Is Delivered to Host Cells in an LFA-1-Indepdendent Manner When Associated With Outer Membrane Vesicles. Toxins (Basel) (2018) 10(10):414. doi: 10.3390/toxins10100414
46. Zingl FG, Thapa HB, Scharf M, Kohl P, Müller AM, Schild S. Outer Membrane Vesicles of Vibrio Cholerae Protect and Deliver Active Cholera Toxin to Host Cells via Porin-Dependent Uptake. mBio (2021) 12(3):e0053421. doi: 10.1128/mBio.00534-21
47. Rubio APD, Martínez J, Palavecino M, Fuentes F, López CMS, Marcilla A, et al. Transcytosis of Bacillus Subtilis Extracellular Vesicles Through an In Vitro Intestinal Epithelial Cell Model. Sci Rep (2020) 10(1):3120. doi: 10.1038/s41598-020-60077-4
48. Han EC, Choi SY, Lee Y, Park JW, Hong SH, Lee HJ. Extracellular RNAs in Periodontopathogenic Outer Membrane Vesicles Promote TNF-α Production in Human Macrophages and Cross the Blood-Brain Barrier in Mice. FASEB J (2019) 33(12):13412–22. doi: 10.1096/fj.201901575R
49. Ha JY, Choi SY, Lee JH, Hong SH, Lee HJ. Delivery of Periodontopathogenic Extracellular Vesicles to Brain Monocytes and Microglial IL-6 Promotion by RNA Cargo. Front Mol Biosci (2020) 7:596366. doi: 10.3389/fmolb.2020.596366
50. Dehinwal R, Cooley D, Rakov AV, Alugupalli AS, Harmon J, Cunrath O, et al. Increased Production of Outer Membrane Vesicles by Salmonella Interferes With Complement-Mediated Innate Immune Attack. mBio (2021) 12(3):e0086921. doi: 10.1128/mBio.00869-21
51. Lekmeechai S, Su YC, Brant M, Alvarado-Kristensson M, Vallström A, Obi I, et al. Helicobacter Pylori Outer Membrane Vesicles Protect the Pathogen From Reactive Oxygen Species of the Respiratory Burst. Front Microbiol (2018) 9:1837. doi: 10.3389/fmicb.2018.01837
52. Vanhove AS, Duperthuy M, Charrière GM, Le Roux F, Goudenège D, Gourbal B, et al. Outer Membrane Vesicles Are Vehicles for the Delivery of Vibrio Tasmaniensis Virulence Factors to Oyster Immune Cells. Environ Microbiol (2015) 17(4):1152–65. doi: 10.1111/1462-2920.12535
53. Kengmo Tchoupa A, Peschel A. Staphylococcus Aureus Releases Proinflammatory Membrane Vesicles To Resist Antimicrobial Fatty Acids. mSphere (2020) 5(5):e00804–20. doi: 10.1128/mSphere.00804-20
54. Singh PP, Li L, Schorey JS. Exosomal RNA From Mycobacterium Tuberculosis-Infected Cells Is Functional in Recipient Macrophages. Traffic (2015) 16(6):555–71. doi: 10.1111/tra.12278
55. Alipoor SD, Mortaz E, Tabarsi P, Farnia P, Mirsaeidi M, Garssen J, et al. Bovis Bacillus Calmette-Guerin (BCG) Infection Induces Exosomal miRNA Release by Human Macrophages. J Transl Med (2017) 15(1):105. doi: 10.1186/s12967-017-1205-9
56. Hare NJ, Chan B, Chan E, Kaufman KL, Britton WJ, Saunders BM. Microparticles Released From Mycobacterium Tuberculosis-Infected Human Macrophages Contain Increased Levels of the Type I Interferon Inducible Proteins Including ISG15. Proteomics (2015) 15(17):3020–9. doi: 10.1002/pmic.201400610
57. Giri PK, Kruh NA, Dobos KM, Schorey JS. Proteomic Analysis Identifies Highly Antigenic Proteins in Exosomes From M. Tuberculosis-Infected and Culture Filtrate Protein-Treated Macrophages. Proteomics (2010) 10(17):3190–202. doi: 10.1002/pmic.200900840
58. Singh PP, Smith VL, Karakousis PC, Schorey JS. Exosomes Isolated From Mycobacteria-Infected Mice or Cultured Macrophages can Recruit and Activate Immune Cells In Vitro and In Vivo. J Immunol (2012) 189(2):777–85. doi: 10.4049/jimmunol.1103638
59. Walters SB, Kieckbusch J, Nagalingam G, Swain A, Latham SL, Grau GE, et al. Microparticles From Mycobacteria-Infected Macrophages Promote Inflammation and Cellular Migration. J Immunol (2013) 190(2):669–77. doi: 10.4049/jimmunol.1201856
60. Koeppen K, Nymon A, Barnaby R, Li Z, Hampton TH, Ashare A, et al. CF Monocyte-Derived Macrophages Have an Attenuated Response to Extracellular Vesicles Secreted by Airway Epithelial Cells. Am J Physiol Lung Cell Mol Physiol (2021) 320(4):L530–l44. doi: 10.1152/ajplung.00621.2020
61. Athman JJ, Wang Y, McDonald DJ, Boom WH, Harding CV, Wearsch PA. Bacterial Membrane Vesicles Mediate the Release of Mycobacterium Tuberculosis Lipoglycans and Lipoproteins From Infected Macrophages. J Immunol (2015) 195(3):1044–53. doi: 10.4049/jimmunol.1402894
62. Prados-Rosales R, Baena A, Martinez LR, Luque-Garcia J, Kalscheuer R, Veeraraghavan U, et al. Mycobacteria Release Active Membrane Vesicles That Modulate Immune Responses in a TLR2-Dependent Manner in Mice. J Clin Invest (2011) 121(4):1471–83. doi: 10.1172/jci44261
63. Park KS, Lee J, Jang SC, Kim SR, Jang MH, Lötvall J, et al. Pulmonary Inflammation Induced by Bacteria-Free Outer Membrane Vesicles From Pseudomonas Aeruginosa. Am J Respir Cell Mol Biol (2013) 49(4):637–45. doi: 10.1165/rcmb.2012-0370OC
64. Kaparakis M, Turnbull L, Carneiro L, Firth S, Coleman HA, Parkington HC, et al. Bacterial Membrane Vesicles Deliver Peptidoglycan to NOD1 in Epithelial Cells. Cell Microbiol (2010) 12(3):372–85. doi: 10.1111/j.1462-5822.2009.01404.x
65. Yang J, Hwang I, Lee E, Shin SJ, Lee EJ, Rhee JH, et al. Bacterial Outer Membrane Vesicle-Mediated Cytosolic Delivery of Flagellin Triggers Host NLRC4 Canonical Inflammasome Signaling. Front Immunol (2020) 11:581165. doi: 10.3389/fimmu.2020.581165
66. Ding FX, Liu B, Zou WJ, Li QB, Tian DY, Fu Z. Pseudomonas Aeruginosa-Derived Exosomes Ameliorates Allergic Reactions via Inducing the T(reg) Response in Asthma. Pediatr Res (2018) 84(1):125–33. doi: 10.1038/s41390-018-0020-1
67. Liu B, Ding F, Cao D, Liu J, Wang Y, Wu C. Pseudomonas Aeruginosa Outer Membrane Vesicles Ameliorates Lung Ischemia-Reperfusion Injury by Regulating the Balance of Regulatory T Cells and Th17 Cells Through Tim-3 and TLR4/NF-κb Pathway. Inflammation Res (2021) 70(8):891–902. doi: 10.1007/s00011-021-01483-w
68. Shimoda A, Ueda K, Nishiumi S, Murata-Kamiya N, Mukai SA, Sawada S, et al. Exosomes as Nanocarriers for Systemic Delivery of the Helicobacter Pylori Virulence Factor CagA. Sci Rep (2016) 6:18346. doi: 10.1038/srep18346
69. Yang S, Xia YP, Luo XY, Chen SL, Li BW, Ye ZM, et al. Exosomal CagA Derived From Helicobacter Pylori-Infected Gastric Epithelial Cells Induces Macrophage Foam Cell Formation and Promotes Atherosclerosis. J Mol Cell Cardiol (2019) 135:40–51. doi: 10.1016/j.yjmcc.2019.07.011
70. Li N, Liu SF, Dong K, Zhang GC, Huang J, Wang ZH, et al. Exosome-Transmitted miR-25 Induced by H. Pylori Promotes Vascular Endothelial Cell Injury by Targeting Klf2. Front Cell Infect Microbiol (2019) 9:366. doi: 10.3389/fcimb.2019.00366
71. Che Y, Geng B, Xu Y, Miao X, Chen L, Mu X, et al. Helicobacter Pylori-Induced Exosomal MET Educates Tumour-Associated Macrophages to Promote Gastric Cancer Progression. J Cell Mol Med (2018) 22(11):5708–19. doi: 10.1111/jcmm.13847
72. Hui WW, Hercik K, Belsare S, Alugubelly N, Clapp B, Rinaldi C, et al. Salmonella Enterica Serovar Typhimurium Alters the Extracellular Proteome of Macrophages and Leads to the Production of Proinflammatory Exosomes. Infect Immun (2018) 86(2):e00386–17. doi: 10.1128/iai.00386-17
73. Hui WW, Emerson LE, Clapp B, Sheppe AE, Sharma J, Del Castillo J, et al. Antigen-Encapsulating Host Extracellular Vesicles Derived From Salmonella-Infected Cells Stimulate Pathogen-Specific Th1-Type Responses In Vivo. PloS Pathog (2021) 17(5):e1009465. doi: 10.1371/journal.ppat.1009465
74. Winter J, Letley D, Rhead J, Atherton J, Robinson K. Helicobacter Pylori Membrane Vesicles Stimulate Innate Pro- and Anti-Inflammatory Responses and Induce Apoptosis in Jurkat T Cells. Infect Immun (2014) 82(4):1372–81. doi: 10.1128/iai.01443-13
75. Chitcholtan K, Hampton MB, Keenan JI. Outer Membrane Vesicles Enhance the Carcinogenic Potential of Helicobacter Pylori. Carcinogenesis (2008) 29(12):2400–5. doi: 10.1093/carcin/bgn218
76. Wang N, Zhou F, Chen C, Luo H, Guo J, Wang W, et al. Role of Outer Membrane Vesicles From Helicobacter Pylori in Atherosclerosis. Front Cell Dev Biol (2021) 9:673993. doi: 10.3389/fcell.2021.673993
77. Guidi R, Levi L, Rouf SF, Puiac S, Rhen M, Frisan T. Salmonella Enterica Delivers Its Genotoxin Through Outer Membrane Vesicles Secreted From Infected Cells. Cell Microbiol (2013) 15(12):2034–50. doi: 10.1111/cmi.12172
78. Yoon H, Ansong C, Adkins JN, Heffron F. Discovery of Salmonella Virulence Factors Translocated via Outer Membrane Vesicles to Murine Macrophages. Infect Immun (2011) 79(6):2182–92. doi: 10.1128/iai.01277-10
79. Losier TT, Akuma M, McKee-Muir OC, LeBlond ND, Suk Y, Alsaadi RM, et al. AMPK Promotes Xenophagy Through Priming of Autophagic Kinases Upon Detection of Bacterial Outer Membrane Vesicles. Cell Rep (2019) 26(8):2150–65.e5. doi: 10.1016/j.celrep.2019.01.062
80. Patras KA, Ha AD, Rooholfada E, Olson J, Ramachandra Rao SP, Lin AE, et al. Augmentation of Urinary Lactoferrin Enhances Host Innate Immune Clearance of Uropathogenic Escherichia Coli. J Innate Immun (2019) 11(6):481–95. doi: 10.1159/000499342
81. Wu Z, Li Y, Liu Q, Liu Y, Chen L, Zhao H, et al. Pyroptosis Engagement and Bladder Urothelial Cell-Derived Exosomes Recruit Mast Cells and Induce Barrier Dysfunction of Bladder Urothelium After Uropathogenic E. Coli Infection. Am J Physiol Cell Physiol (2019) 317(3):C544–c55. doi: 10.1152/ajpcell.00102.2019
82. Kouokam JC, Wai SN, Fällman M, Dobrindt U, Hacker J, Uhlin BE. Active Cytotoxic Necrotizing Factor 1 Associated With Outer Membrane Vesicles From Uropathogenic Escherichia Coli. Infect Immun (2006) 74(4):2022–30. doi: 10.1128/iai.74.4.2022-2030.2006
83. Davis JM, Carvalho HM, Rasmussen SB, O'Brien AD. Cytotoxic Necrotizing Factor Type 1 Delivered by Outer Membrane Vesicles of Uropathogenic Escherichia Coli Attenuates Polymorphonuclear Leukocyte Antimicrobial Activity and Chemotaxis. Infect Immun (2006) 74(8):4401–8. doi: 10.1128/iai.00637-06
84. Davis JM, Rasmussen SB, O'Brien AD. Cytotoxic Necrotizing Factor Type 1 Production by Uropathogenic Escherichia Coli Modulates Polymorphonuclear Leukocyte Function. Infect Immun (2005) 73(9):5301–10. doi: 10.1128/iai.73.9.5301-5310.2005
85. Cho SM, Shin S, Kim Y, Song W, Hong SG, Jeong SH, et al. A Novel Approach for Tuberculosis Diagnosis Using Exosomal DNA and Droplet Digital PCR. Clin Microbiol Infect (2020) 26(7):942. doi: 10.1016/j.cmi.2019.11.012
86. Herrmann IK, Bertazzo S, O'Callaghan DJ, Schlegel AA, Kallepitis C, Antcliffe DB, et al. Differentiating Sepsis From Non-Infectious Systemic Inflammation Based on Microvesicle-Bacteria Aggregation. Nanoscale (2015) 7(32):13511–20. doi: 10.1039/c5nr01851j
87. Mizutani K, Kawakami K, Horie K, Fujita Y, Kameyama K, Kato T, et al. Urinary Exosome as a Potential Biomarker for Urinary Tract Infection. Cell Microbiol (2019) 21(7):e13020. doi: 10.1111/cmi.13020
88. Hao Q, Gudapati V, Monsel A, Park JH, Hu S, Kato H, et al. Mesenchymal Stem Cell-Derived Extracellular Vesicles Decrease Lung Injury in Mice. J Immunol (2019) 203(7):1961–72. doi: 10.4049/jimmunol.1801534
89. Yang X, Shi G, Guo J, Wang C, He Y. Exosome-Encapsulated Antibiotic Against Intracellular Infections of Methicillin-Resistant Staphylococcus Aureus. Int J Nanomedicine (2018) 13:8095–104. doi: 10.2147/ijn.S179380
90. Qian Z, Bai Y, Zhou J, Li L, Na J, Fan Y, et al. A Moisturizing Chitosan-Silk Fibroin Dressing With Silver Nanoparticles-Adsorbed Exosomes for Repairing Infected Wounds. J Mater Chem B (2020) 8(32):7197–212. doi: 10.1039/d0tb01100b
91. Song Z, Li B, Zhang Y, Li R, Ruan H, Wu J, et al. Outer Membrane Vesicles of Helicobacter Pylori 7.13 as Adjuvants Promote Protective Efficacy Against Helicobacter Pylori Infection. Front Microbiol (2020) 11:1340. doi: 10.3389/fmicb.2020.01340
92. Liu Q, Tan K, Yuan J, Song K, Li R, Huang X, et al. Flagellin-Deficient Outer Membrane Vesicles as Adjuvant Induce Cross-Protection of Salmonella Typhimurium Outer Membrane Proteins Against Infection by Heterologous Salmonella Serotypes. Int J Med Microbiol (2018) 308(7):796–802. doi: 10.1016/j.ijmm.2018.06.001
93. Prior JT, Davitt C, Kurtz J, Gellings P, McLachlan JB, Morici LA. Bacterial-Derived Outer Membrane Vesicles Are Potent Adjuvants That Drive Humoral and Cellular Immune Responses. Pharmaceutics (2021) 13(2):131. doi: 10.3390/pharmaceutics13020131
94. Rollier CS, Dold C, Marsay L, Linder A, Green CA, Sadarangani M, et al. Human B Cell Responses to Dominant and Subdominant Antigens Induced by a Meningococcal Outer Membrane Vesicle Vaccine in a Phase I Trial. mSphere (2022) 7(1):e0067421. doi: 10.1128/msphere.00674-21
95. Schetters STT, Jong WSP, Horrevorts SK, Kruijssen LJW, Engels S, Stolk D, et al. Outer Membrane Vesicles Engineered to Express Membrane-Bound Antigen Program Dendritic Cells for Cross-Presentation to CD8(+) T Cells. Acta Biomater (2019) 91:248–57. doi: 10.1016/j.actbio.2019.04.033
96. Zurita ME, Wilk MM, Carriquiriborde F, Bartel E, Moreno G, Misiak A, et al. A Pertussis Outer Membrane Vesicle-Based Vaccine Induces Lung-Resident Memory CD4 T Cells and Protection Against Bordetella Pertussis, Including Pertactin Deficient Strains. Front Cell Infect Microbiol (2019) 9:125. doi: 10.3389/fcimb.2019.00125
97. Petousis-Harris H, Paynter J, Morgan J, Saxton P, McArdle B, Goodyear-Smith F, et al. Effectiveness of a Group B Outer Membrane Vesicle Meningococcal Vaccine Against Gonorrhoea in New Zealand: A Retrospective Case-Control Study. Lancet (2017) 390(10102):1603–10. doi: 10.1016/s0140-6736(17)31449-6
98. Gujrati V, Kim S, Kim SH, Min JJ, Choy HE, Kim SC, et al. Bioengineered Bacterial Outer Membrane Vesicles as Cell-Specific Drug-Delivery Vehicles for Cancer Therapy. ACS Nano (2014) 8(2):1525–37. doi: 10.1021/nn405724x
99. Huang W, Shu C, Hua L, Zhao Y, Xie H, Qi J, et al. Modified Bacterial Outer Membrane Vesicles Induce Autoantibodies for Tumor Therapy. Acta Biomater (2020) 108:300–12. doi: 10.1016/j.actbio.2020.03.030
100. Li Y, Zhao R, Cheng K, Zhang K, Wang Y, Zhang Y, et al. Bacterial Outer Membrane Vesicles Presenting Programmed Death 1 for Improved Cancer Immunotherapy via Immune Activation and Checkpoint Inhibition. ACS Nano (2020) 14(12):16698–711. doi: 10.1021/acsnano.0c03776
101. Kuerban K, Gao X, Zhang H, Liu J, Dong M, Wu L, et al. Doxorubicin-Loaded Bacterial Outer-Membrane Vesicles Exert Enhanced Anti-Tumor Efficacy in Non-Small-Cell Lung Cancer. Acta Pharm Sin B (2020) 10(8):1534–48. doi: 10.1016/j.apsb.2020.02.002
102. Yekula A, Minciacchi VR, Morello M, Shao H, Park Y, Zhang X, et al. Large and Small Extracellular Vesicles Released by Glioma Cells In Vitro and In Vivo. J Extracell Vesicles (2020) 9(1):1689784. doi: 10.1080/20013078.2019.1689784
103. Jeppesen DK, Fenix AM, Franklin JL, Higginbotham JN, Zhang Q, Zimmerman LJ, et al. Reassessment of Exosome Composition. Cell (2019) 177(2):428–45.e18. doi: 10.1016/j.cell.2019.02.029
104. Luo P, Mao K, Xu J, Wu F, Wang X, Wang S, et al. Metabolic Characteristics of Large and Small Extracellular Vesicles From Pleural Effusion Reveal Biomarker Candidates for the Diagnosis of Tuberculosis and Malignancy. J Extracell Vesicles (2020) 9(1):1790158. doi: 10.1080/20013078.2020.1790158
105. Bordanaba-Florit G, Royo F, Kruglik SG, Falcón-Pérez JM. Using Single-Vesicle Technologies to Unravel the Heterogeneity of Extracellular Vesicles. Nat Protoc (2021) 16(7):3163–85. doi: 10.1038/s41596-021-00551-z
106. Jung AL, Herkt CE, Schulz C, Bolte K, Seidel K, Scheller N, et al. Legionella Pneumophila Infection Activates Bystander Cells Differentially by Bacterial and Host Cell Vesicles. Sci Rep (2017) 7(1):6301. doi: 10.1038/s41598-017-06443-1
Keywords: extracellular vesicle (EV), bacteria, host, host-bacteria interaction, immune response
Citation: Zou C, Zhang Y, Liu H, Wu Y and Zhou X (2022) Extracellular Vesicles: Recent Insights Into the Interaction Between Host and Pathogenic Bacteria. Front. Immunol. 13:840550. doi: 10.3389/fimmu.2022.840550
Received: 21 December 2021; Accepted: 27 April 2022;
Published: 25 May 2022.
Edited by:
Leonardo Nimrichter, Federal University of Rio de Janeiro, BrazilReviewed by:
Subhash Chand, University of Nebraska Medical Center, United StatesMaurizio Muraca, University of Padua, Italy
Copyright © 2022 Zou, Zhang, Liu, Wu and Zhou. This is an open-access article distributed under the terms of the Creative Commons Attribution License (CC BY). The use, distribution or reproduction in other forums is permitted, provided the original author(s) and the copyright owner(s) are credited and that the original publication in this journal is cited, in accordance with accepted academic practice. No use, distribution or reproduction is permitted which does not comply with these terms.
*Correspondence: Xikun Zhou, xikunzhou@scu.edu.cn
†These authors have contributed equally to this work and share first authorship