- 1Department of Neurology, Klinikum rechts der Isar, Technical University of Munich, Munich, Germany
- 2Department of Neurology, University Hospital Erlangen, Friedrich–Alexander University Erlangen–Nürnberg, Erlangen, Germany
Astrocytes play important roles in numerous central nervous system disorders including autoimmune inflammatory, hypoxic, and degenerative diseases such as Multiple Sclerosis, ischemic stroke, and Alzheimer’s disease. Depending on the spatial and temporal context, activated astrocytes may contribute to the pathogenesis, progression, and recovery of disease. Recent progress in the dissection of transcriptional responses to varying forms of central nervous system insult has shed light on the mechanisms that govern the complexity of reactive astrocyte functions. While a large body of research focuses on the pathogenic effects of reactive astrocytes, little is known about how they limit inflammation and contribute to tissue regeneration. However, these protective astrocyte pathways might be of relevance for the understanding of the underlying pathology in disease and may lead to novel targeted approaches to treat autoimmune inflammatory and degenerative disorders of the central nervous system. In this review article, we have revisited the emerging concept of protective astrocyte functions and discuss their role in the recovery from inflammatory and ischemic disease as well as their role in degenerative disorders. Focusing on soluble astrocyte derived mediators, we aggregate the existing knowledge on astrocyte functions in the maintenance of homeostasis as well as their reparative and tissue-protective function after acute lesions and in neurodegenerative disorders. Finally, we give an outlook of how these mediators may guide future therapeutic strategies to tackle yet untreatable disorders of the central nervous system.
Introduction
Astrocytes are the most abundant cell type in the mammalian central nervous system (CNS) and responsible for a multitude of functions. During development, astrocytes arise from neural stem cells (NSCs) in the subventricular zone (SVZ) and migrate along radial glia processes to populate the CNS (1). Once their migration is complete, astrocytes differentiate into subgroups with a high degree of functional and regional specialization (1–6). During postnatal development, astrocytes instruct the formation of excitatory and inhibitory synapses, support developmental myelination, and aid the establishment of complex neural circuitry through the secretion of soluble factors (7–10) and physical cell contact (11–13). Throughout adulthood, astrocytes form close interactions with neurons to provide structural support and engage in metabolic coupling, serving as nutrient source and storage for neurons (Figures 1A, B) (14). Particularly lactate produced by astrocytes has been demonstrated to play an important role in the modulation of neuronal excitability and plasticity (15). Furthermore, astrocytes actively take part in synaptic transmission and have been shown to modulate cognitive functions through the clearance of neurotransmitter and other extracellular factors (16–20) (Figure 1B). In this context, the inward rectifying K+ channel Kir4.1 has gained attention as part of a K+ spatial buffering system that is required for neuronal transmission and functioning (Figure 1B). Kir4.1 is highly expressed in astrocytic endfeet, and its misregulation has been linked to numerous neurological disorders (21–23).
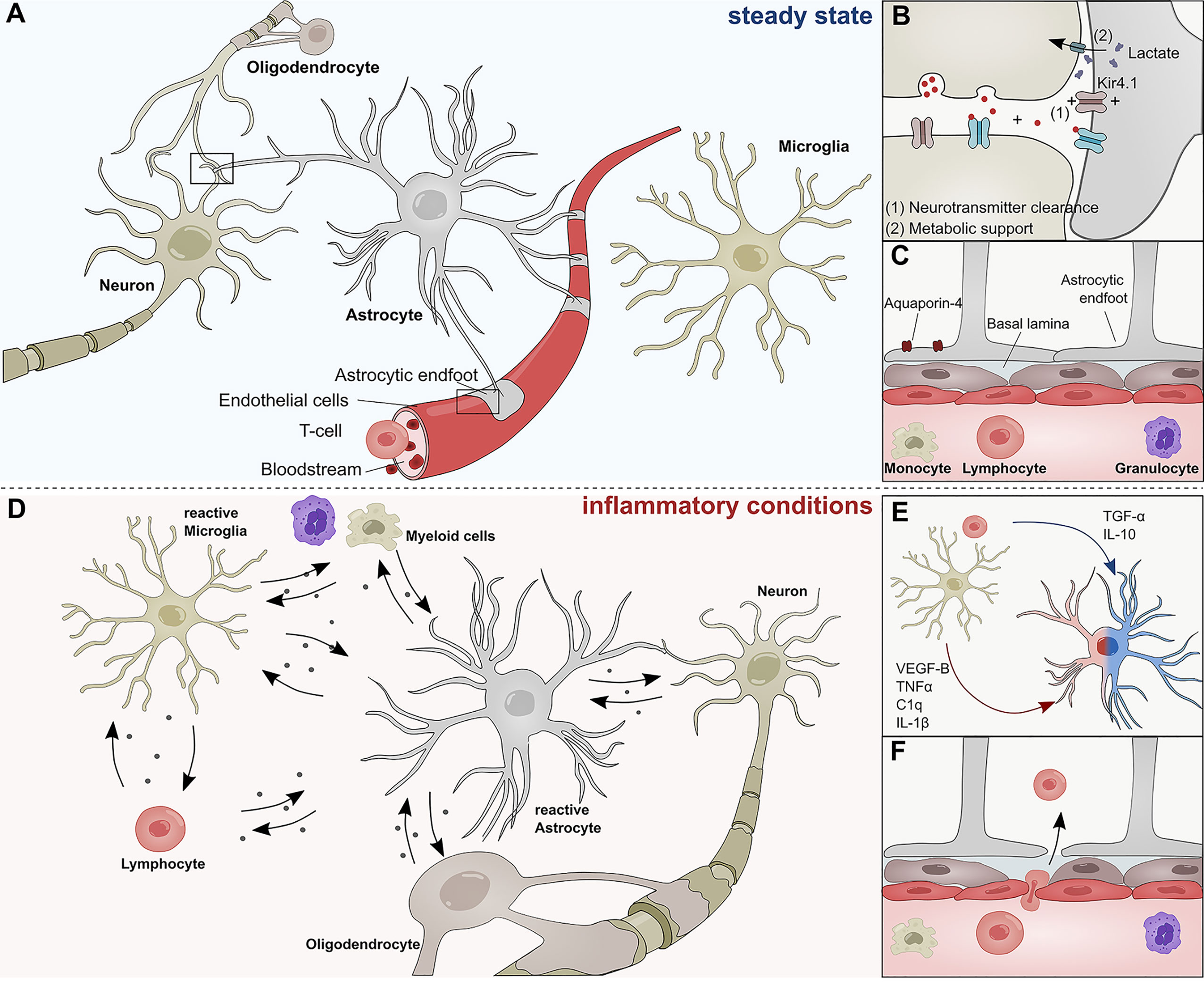
Figure 1 Role of astrocytes in the steady state and inflammatory conditions. (A) Astrocytes interact with neurons, oligodendrocytes, microglia, and cells of the BBB during steady state conditions. (B) Astrocytes form tripartite synapses with neurons and regulate their synaptic transmission through metabolic support and the clearance of neurotransmitters. (C) Astrocytic endfeet line the cerebral vasculature and are a constituent of the blood brain barrier, thus limiting the infiltration of pathogens and peripheral immune cells into the central nervous system. Their endfeet express high levels of Aqp-4 and form a close interaction with pericytes and the basal lamina of the brain parenchyma. (D) During inflammatory conditions, reactive astrocyte secrete a plethora of inflammatory mediators that regulate functions of myeloid cells, lymphocytes, oligodendrocytes, neurons, and microglia. (E) Soluble inflammatory mediators derived from mircoglia and other immune cells differentially induce pathogenic (red) or protective (blue) astrocyte functions. (F) Peripheral immune cells pervade the BBB during inflammatory conditions and transgress into the CNS. C1q, Complement component 1q; IL-1β, Interleukin-1 β; IL-10, Interleukin 10; TNF-α, Tumor necrosis factor α; TGF-α, Transforming growth factor α; VEGF-B, Vascular endothelial growth factor B.
Besides their versatile role during neurogenesis and their contribution to the maintenance of neuronal circuitry, astrocytes are key participants in the formation and maintenance of the blood brain barrier (BBB) (24, 25) (Figure 1C). During CNS angiogenesis, astrocytes extend their polarized endfeet around the abluminal side of cerebral blood vessels and aid early sprout guidance and maturation by the expression of transporters, anti-permeability proteins and the secretion of growth factors (24–26). As key constituent of the glial limitans, astrocytic endfeet line the basement membrane surrounding the cerebral vasculature and provide a physical barrier between CNS and the peripheral blood system, thus limiting the influx of pathogens and large hydrophilic molecules (Figure 1C) (24, 25, 27–29). Furthermore, astrocytes control water homeostasis in the CNS via Aquaporin-4 (Aqp4) and other channel proteins involved in bidirectional fluid exchange across the BBB (30) (Figure 1B). The importance of Aqp4 in the CNS is demonstrated in a series of publications that link the (mal-)function of Aqp4 to multiple neurological disorders (31–34). Aqp4 has also been identified as a major target of autoantibodies in patients suffering from neuromyelitis optica (NMO), a rare CNS inflammatory disorder that has historically been closely associated to MS (35).
In addition to their versatile functions in the steady-state, astrocytes sense and react to danger signals in a multistep process referred to as astrogliosis (36, 37). Combinatorial exposure to a broad spectrum of extracellular cues, including cytokines, growth factors, and hormones induces transcriptional remodeling, resulting in cellular hypertrophy, proliferation and secretion of inflammatory mediators (Figure 1D) (36). The severity and permanence of these transcriptional changes is dependent on the type and strength of the stimuli and can range from reversible alterations to severe astrogliosis with compact scar formation (36, 38). Most forms of astrogliosis share the upregulation of glial fibrillary acidic protein (GFAP), a phenomenon that has been observed in multiple CNS disorders (1, 39–41).
For many decades, it was believed that severe astrogliosis and the formation of a glial scar inhibits axonal re-growth and is detrimental for neurological outcome. However, an increasing amount of evidence suggests that astrocytes also play beneficial roles in disease (42, 43). Methodological advances in the genomic analysis of reactive astrocytes have begun to shed light on the molecular mechanisms that define the fine line between pathogenic and protective astrocyte functions. For instance, a landmark study by Zamanian and colleagues (44) demonstrated that astrocytes respond differentially to varying forms of CNS insult. While exposure to lipopolysaccharide (LPS) resulted in the upregulation of pro-inflammatory genes and skewed astrocytes toward a cytotoxic profile, ischemia induced transcriptional programs that are associated with neuroprotective functions (44–47). In this context, particularly intercellular crosstalk with microglia has been identified as key regulator of astrocyte functions. Work by several groups including ours has unraveled molecular mechanisms through which microglia-derived molecules such as interleukin (IL)-1β, IL-10, tumor necrosis factor (TNF)-α, vascular endothelial growth factor (VEGF)-B, or transforming growth factor (TGF)- α, among others, modulate transcriptional programs in astrocytes that are associated to degenerative or protective functions (Figure 1E) (45, 48). In addition to microglia, numerous other CNS-resident and non-CNS-resident cell types modulate astroglial properties and are themselves subject to factors secreted by reactive astrocytes under inflammatory conditions (49). For instance, reactive astrocytes use contact- and diffusion-mediated mechanisms to modulate trafficking of peripheral immune cells into the CNS, a topic that has been extensively reviewed by Sofroniew and others (25, 28) (Figure 1F). Once the peripheral cells have extravasated, they accumulate in perivascular spaces where they are in close contact to astrocytic endfeet (50). It is possible that during this stage, MHCII+ astrocytes function as antigen-presenting cells to reactivate infiltrating lymphocytes and promote inflammation (51–53). Furthermore, there is increasing evidence that astrocytes control the survival of T-cells and B-cells via co-regulatory and secreted factors. Indeed, while FasL expression by astrocytes induces cell death in infiltrating lymphocytes, B cell–activating factor of the tumor necrosis factor (TNF) family (BAFF) produced by astrocytes promotes B-cell survival in inflammatory conditions and primary B cell lymphoma (54–56). Interestingly, astrocytes themselves respond to myeloid-derived APRIL, another member of the TNF superfamily with an increase in IL-10 production, consequently suppressing pro-inflammatory T-cell functions (57). These interactions between reactive astrocytes and cells of the adaptive immune system are complemented by their functions as part of the cerebral innate immune system (58).
Another degree of complexity is added when analyzing the temporal dynamics of astrogliosis in the context of disease. In vivo ablation experiments of astrocytes in experimental autoimmune encephalomyelitis (EAE), an animal model of Multiple Sclerosis (MS), demonstrated that astrocytes are required for disease suppression in early EAE stages, as loss of astrocytes worsened disease, characterized by increased BBB permeability, leukocyte infiltration, and neuronal death (59–62). Conversely, selective ablation of reactive astrocytes during the chronic phase of EAE ameliorated disease, marked by decreased microglial activation and monocyte infiltration (60). This and other studies underline the dire need to further dissect the contribution of astrocytes to the pathogenesis and progression of numerous CNS disorders.
While many studies focus on the pathogenic potential of reactive astrocytes, molecular mechanisms underlying their protective effects remain elusive at large. Here, we will discuss astrocyte-derived mediators with anti-inflammatory or tissue-protective properties, and examine how these factors may guide future therapeutic strategies. In this context we will not focus on protective astrocyte functions mediated by inflammatory cytokines or cell-cell contact, which have been reviewed extensively elsewhere (49, 63, 64), but rather concentrate on soluble factors often overlooked in the field of neuroinflammation.
Protective Effects of Reactive Astrocytes Following CNS Insult
A widely recognized protective function of astrogliosis is the formation of a physical barrier, which limits the influx of peripheral immune cells and thus restricts lesion size (28, 65–67). This function has been discussed in depth in a series of excellent reviews (24, 25, 28, 37, 63). Here, we will focus on astrocyte secreted mediators relevant for astrocyte protective functions. Advances in single cell sequencing, spatial transcriptomics, and conditional knock-down approaches demonstrate that reactive astrocytes secrete a plethora of anti-inflammatory and tissue-protective mediators that act on numerous cells to control their inflammatory state (Table 1). This review will focus on three major domains to summarize the existing knowledge on astrocyte protective function: neurotrophic factors, neuropoetic cytokines, and growth factors.
Neurotrophic Factors
Neurotrophic factors (NTFs) play an essential role in the growth, differentiation, and survival of neurons in health and disease. They can broadly be divided into neurotrophins, members of the ciliary neurotrophic factor (CNTF) family, and members of the glia derived neurotrophic factor (GDNF) family. While their role in the survival of neurons is relatively well defined, little is known about inflammatory functions and how astrocytes contribute to their production. Generally, glial cells are known to express low levels of NTFs under homeostatic conditions, but significantly upregulate their production following CNS damage (109, 110).
BDNF
Brain-derived neurotrophic factor (BDNF) and nerve growth factor (NGF) are members of the neurotrophin family and highly expressed by astrocytes during development (109, 111–113). Throughout adulthood, astrocytes express low levels of BDNF but significantly upregulate its production in response to spinal cord injury (SCI) (114, 115), ischemia (115), and neuroinflammation (68, 69). BDNF signals through two receptors, the high-affinity TrkB receptor and the low-affinity p75NTR receptor, both of which are expressed throughout the CNS by neurons, astrocytes, and oligodendrocytes (116). While BDNF/TrkB signaling on neurons has been shown to promote survival and neurite outgrowth (117), p75NTR signaling induces apoptosis in cultured neurons (118). This dualistic signaling system corresponds to the dichotomic effector functions of BDNF. Early studies in the context of axotomy and SCI demonstrated beneficial effects of BDNF on the regeneration and long-term survival of neurons (119, 120) (Figure 2). In EAE, reports suggest that BDNF depletion in CNS resident cells during the initial phase worsens disease, while deletion during later stages does not lead to significant differences (121). Although this protective effect might depend on multiple cell types, astrocytes have been suggested to be a key participant in BDNF-dependent remyelination in the cuprizone model of de- and remyelination (68). This is supported by observations of increased progenitor cell proliferation and maturation of neurons following lentiviral overexpression of Bdnf in hippocampal astrocytes (70) (Figure 2). Furthermore, a study by Linker and colleagues (69) demonstrated that conditional depletion of BDNF in astrocytes worsens EAE severity. Interestingly, the authors did not observe changes in infiltrating immune cells, but demonstrated a significant increase in axonal loss and demyelination.
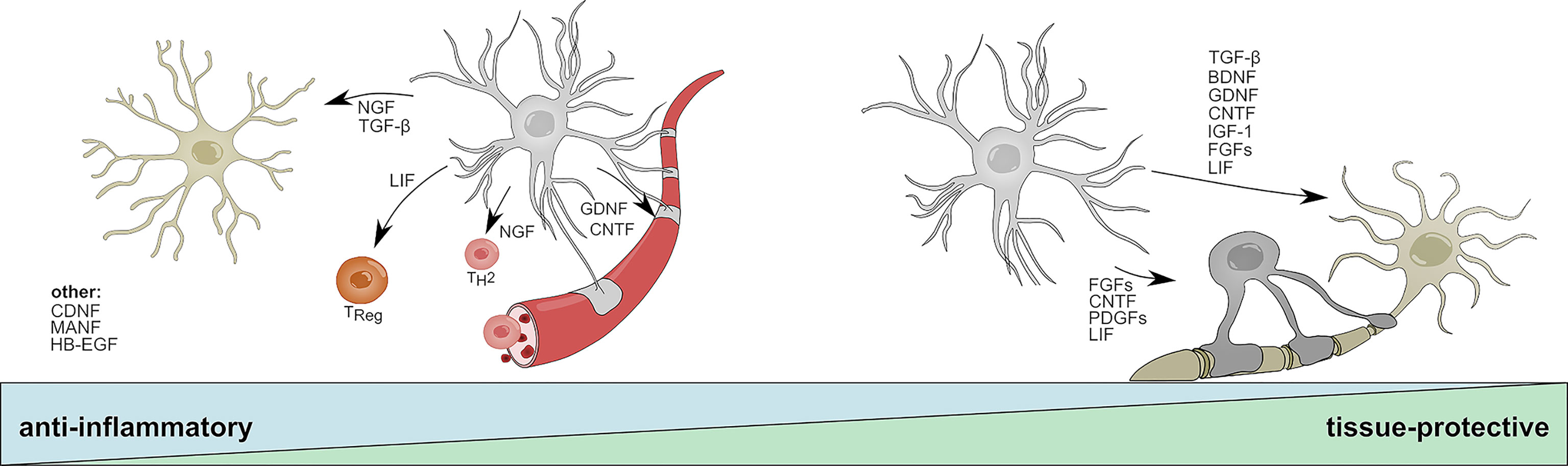
Figure 2 Anti-inflammatory and tissue-protective functions of reactive astrocytes. Activated astrocytes secrete soluble mediators with anti-inflammatory functions that help to resolve acute inflammation following CNS insult. NGF and TGF-β promote beneficial functions in microglia; LIF skews CD4 T-cells towards a regulatory phenotype; NGF promotes the differentiation into TH2 cells; GDNF and CNDF have beneficial effects on blood brain barrier permeability. CNDF, MANF, and HB-EGF have been associated to anti-inflammatory functions on multiple cell types or cells that are not displayed. During later stages, astrocyte-derived mediators promote the survival of neurons and oligodendrocytes and aid the long-term regeneration following CNS insult. TGF-β, BDNF, FGF family members, DNF, CNTF, IGF-1, and LIF increase neuronal survival; CNTF, LIF, and PDGF family members promote oligodendrocyte differentiation and myelination. BDNF, Brain derived neurotrophic factor; CNTF, Ciliary neurotrophic factor; FGF, Fibroblast growth factor; GDNF, Glial cell line-derived neurotrophic factor; HB-EGF, Heparin-binding epidermal growth factor; IGF-1, Insulin-like growth factor 1; LIF, Leukemia inhibitory factor; MANF, Mesencephalic astrocyte-derived neurotrophic factor; NGF, Nerve growth factor; PDGF, Platelet-derived growth factor; TH2, T helper type 2 cell; TReg, T regulatory cell; TGF-β, Transforming growth factor β.
Overall, these findings suggest that BDNF regulates axonal myelination and neuronal function through Trk/p75NTR signaling on neurons and potentially oligodendrocytes, making it a key constituent of neuronal health. This also becomes clear in the context of multiple neurodegenerative disorders, where a single nucleotide polymorphism (SNP) in the BDNF gene is associated to increased susceptibility, incidence and severity of MS (122–124) and Alzheimer’s disease (AD) (125), correlating with cognitive dysfunction (126, 127). Although the relative contribution of BDNF producing cells to the protective effects of BDNF remains under debate, a substantial body of evidence points to astrocytes as key drivers of BDNF mediated effects in disease. Furthermore, astrocyte-derived BDNF has been identified as mediator of the therapeutic functions of glatiramer acetate (GA), a FDA-approved drug for the treatment of relapse-remitting MS (RRMS) in a mouse model of neurodegeneration, demonstrating the potential of astrocyte-derived BDNF for future therapeutic strategies (128). Nonetheless, recent evidence suggest that there is a fine line between protective and pathogenic astrocyte-mediated functions of BDNF, as astrocytes themselves respond to increased levels of BDNF with the secretion of neurotoxic amounts of nitric oxide (NO), demonstrating a sophisticated feedback loop that prevents excessive BDNF signaling (129).
NGF
In contrast to BDNF, little is known about the immunomodulatory and tissue-protective functions of astrocyte-derived NGF. Early studies suggest upregulation of Ngf mRNA in astrocytes in models of traumatic injury, Parkinson’s disease (PD), and neuroinflammation (130–132). Similar to BDNF, mature NGF is cleaved from its precursor pro-NGF and signals via a dual receptor system consisting of TrkA and p75NTR (133). While mature NGF preferentially binds to TrkA and promotes neuronal survival, pro-NGF has a higher affinity to p75NTR and has been shown to induce apoptotic signaling in oligodendrocytes and neurons (134, 135). Under homeostatic conditions, pro-NGF and its mature form signal synergistically through TrkA/p75NTR to promote the survival and differentiation of neuronal cells; however, imbalances in the relative abundances of TrkA and p75NTR have been described in multiple CNS disorders (133, 136–138). Interestingly, several reports demonstrate that activated astrocytes secrete increased amounts of neurotoxic pro-NGF in vitro and following SCI, suggesting a tissue-destructive role of endogenous, astrocyte-derived pro-NGF (71, 72). In contrast, treatment with exogenous NGF has been shown to be beneficial in models of traumatic injury and neuroinflammation (73, 74). For instance, administration of human NGF into the ventricle of marmoset monkeys prevented the development of lesions in an EAE model by skewing infiltrating T-cells towards an anti-inflammatory TH2 phenotype (73) (Figure 2). In line with this observation, a recent study reports that NGF instructs TrkA-mediated phagocytosis of neurotoxic Amyloid-β plaques by microglia in a mouse model of AD (74) (Figure 2). While it remains to be seen, which impact astrocyte-derived NGF has on the net effect of NGF, the activation of astrocytes and subsequent induction of Ngf expression by inflammation or stress-related events may contribute to both beneficial and harmful effects of NGF, depending on the availability of pro-NGF vs. mature NGF and the presence of TrkA vs. p75NTR receptors on adjacent cells (72, 139). Of note, in addition to effects mediated by astrocyte derived NGF, a suppressive function of exogenously administered NGF in reactive astrocytes has been proposed, implicating a potential autocrine feedback loop that limits excessive astroglial activation (140).
GDNF
The GDNF family of neurotrophic factors consists of GDNF, neurturin (NTRN), artemin (ARTN), and persepin (PSPN) (141). All four members belong to the TGF-β superfamily and signal through the RET Tyrosine kinase to regulate the differentiation and survival of multiple distinct sets of neurons (141, 142). Interestingly, alternative signaling receptors, such as the neural cell adhesion molecule (NCAM) have been proposed and numerous studies suggest synergistic signaling with NGF, BDNF, and TGF-β (143–147). Reactive astrocytes rapidly upregulate the production of GDNF in response to LPS, IL-1β, IFN-γ and microglia-derived TNF-α, and have been shown to promote the survival of dopaminergic neurons in vitro (75, 76). This is in line with in vivo studies that demonstrate beneficial effects of astrocyte-specific overexpression of Gdnf in models of Parkinson’s disease (PD) (77, 78). Furthermore, transplantation of human NPCs committed to a glial fate that have been genetically engineered to overexpress GDNF promoted neuronal survival and regeneration in primate models of amyotrophic lateral sclerosis (ALS) (148, 149) (Figure 2). Besides the supportive effects of astrocyte-derived GDNF on neurons, GDNF/GDNFRα signaling has been shown to promote the trans-endothelial resistance in an in vitro BBB model, suggesting a positive effect of astrocyte-derived GDNF on tight-junction function and BBB permeability during neuroinflammation (79) (Figure 2). Collectively, further investigation into the anti-inflammatory and tissue-protective effects of astrocyte-derived GDNF is needed, but given the synergistic signaling of GDNF in combination with TGF-β and other NTFs, astrocytic GDNF may contribute to the reduction of inflammation and regenerative capacities following CNS insult.
CNTF
CNTF composes a separate family of neurotrophic factors and has been extensively studied as inducer of neuronal differentiation, survival and neurite outgrowth (150). Besides its effect on neurons, CNTF has been shown to support the maturation of oligodendrocytes and astrocytes (151–154) (Figure 2). It signals through a heterotrimeric receptor complex consisting of the non-signaling subunit ciliary neurotrophic factor receptor alpha (CNTFRα), and the two signaling chains glycoprotein-130 (gp130) and leukemia inhibitor factor receptor (LIFRβ), which are shared with the distantly related leukemia inhibitory factor (LIF) and interleukin-6 (IL-6) (155). Upon CNTF binding, heterodimerization of gp130 and LIFRβ induces JAK/STAT dependent transcriptional programs that are associated with the differentiation and survival of neurons (156). Under homeostatic conditions, the expression of low levels of Cntf in astrocytes is limited to the white matter, indicating region-specific effects on distinct neuronal subpopulations (157). Interestingly, this finding is concordant with observations of increased Cntf expression in astrocytes and the upregulation of CNTFRα on neurons located in white matter lesions of MS patients (158). A study investigating the spatial and temporal dynamics of multiple NTFs in a cuprizone model of demyelination suggests that astrocytes express CNTF in a biphasic manner during initial demyelination and remyelination (159). Mechanistically, it has been proposed that loss of physical interaction between astrocytes and neurons following injury induces STAT3-mediated Cntf expression in astrocytes, which promotes survival of neurons and oligodendrocytes and may counteract TNF-α induced myelin disintegration during EAE (81–83) (Figure 2). Similar to GDNF, beneficial effects on BBB permeability and a reduction of immune cell infiltrates have been observed following administration of exogenous CNTF in a mouse model of neuroinflammation (160) (Figure 2). Collectively, the current data indicates that astrocyte derived CNTF might contribute to the reduction of acute inflammation and increases the survival of neurons and oligodendrocytes in the context of CNS insult. In addition, CNTF may promote the activation of surrounding astrocytes in an autocrine/paracrine manner.
MANF/CNDF
Mesencephalic Astrocyte-Derived Neurotrophic Factor (MANF) and Cerebral Dopamine Neurotrophic Factor (CDNF) constitute a novel, evolutionary conserved family of NTFs with regenerative capacities in health and disease. Although MANF and CDNF have been originally identified to provide neurotrophic support for dopaminergic neurons, it has become clear that their functions extend beyond those of classical NTFs (161–168). Both NTFs have been associated to numerous tissue-protective and anti-inflammatory functions in models of PD, ischemia and nerve injury (162–164, 168, 169). In addition, a series of recent studies demonstrated that MANF and CDNF are partially retained within the endoplasmic reticulum (ER), where they sense and respond to ER stress by negatively regulating NF-κB dependent inflammatory programs (84, 165, 169–174). In astrocytes, upregulation of both MANF and CDNF has been observed in response to ER stress and experimental stroke, where they alleviate the secretion of pro-inflammatory cytokines IL-1β, TNF-α, and IL-6 (84–86). This is supported by a study using astrocyte-specific overexpression of Manf, which resulted in a downregulation of pro-inflammatory cytokines (84). Taken together, this indicates that astrocytic MANF and CDNF function as cell-autonomous safety switch that prevents ER stress induced overactivation and provides neurotrophic support for neurons (Figure 2). Evidence from a Drosophila model of retinal tissue damage further suggests that MANF counteracts the pro-inflammatory functions of VEGF–related factor 1 (Pvf-1) homologue and is required for successful tissue repair (169). This is of particular interest in the context of glial communication, as VEGF secreted by microglia has been demonstrated to induce pro-inflammatory signaling in astrocytes, and the successive MANF secretion by astrocytes may present an anti-inflammatory mechanism that counteracts pathogenic VEGF signaling (48).
Growth Factors and Neuropoietic Cytokines
PDGF Family Members
Platelet-derived growth factors (PDGFs) and their cognate receptors compose a signaling network that consists of five ligand-dimers (PDGF-AA, PDGF-BB, PDGF-AB, PDGF-CC, PDGF-DD) and three receptors (PDGFR-αα, PDGFR-ββ, PDGFR-αβ) (175). While PDGFs have originally been identified as growth factor for smooth muscle cells (176), they are nowadays viewed as potent inducer of oligodendrocyte proliferation and differentiation (177, 178). Interestingly, the PDGF family of cysteine-knot growth factors also includes members of the VEGF subfamily, of which VEGF-B has been shown to induce pro-inflammatory gene expression in astrocytes (48). Similarly, astrocytes can also respond to PDGF-A and PDGF-C by expression of PDGFR-α, which serves as mitogen and inducer of astrocytic branching (179, 180). In addition, a series of studies demonstrated that astrocytes express PDGF-A and PDGF-B monomers, but not PDGF-C or PDGF-D (181–185). In the developing brain, these astrocyte-derived PDGF variants modulate the proliferation and differentiation of oligodendrocyte precursor cells (OPCs) (184, 186) and potentially regulate the proliferation and survival of neurons (187, 188). In the adult CNS, it remains unclear to what extent astrocytes contribute to the PDGF signaling network, as neurons have also been proposed as source of PDGF-A and PDGF-B (189–191). Nevertheless, early work by Silberstein et al. (182) indicates that cultured astrocytes upregulate the expression of PDGFs in response to TNF-α and TGF-β, suggesting a role of PDGF signaling in inflammatory conditions. In this context, two independent studies investigated the therapeutic effects of astrocyte-derived PDGF-A by conditional overexpression in mouse models of chronic and acute CNS demyelination and revealed that elevated expression of PDGF-A by astrocytes significantly increased OPC survival and population density (87, 88) (Figure 2). While these findings may prove useful to address the progressing demyelination in primary and secondary progressive MS and other degenerative CNS pathologies, important questions remain outstanding. Which programs control the expression of PDGFs in astrocytes? To what extent do astrocyte-derived PDGFs modulate the functions of oligodendrocytes and neurons? And what is their role in the remyelinating brain? Further research into the basic mechanisms of CNS intrinsic signaling of PDGFs is needed to warrant success in their use as future therapeutic target.
FGF Family Members
Fibroblast growth factors (FGFs) constitute a family of at least 20 secreted ligands with pleiotropic roles in the developing and mature CNS (192–203). Most FGF receptors (FGFRs) can respond to multiple FGF ligands (e.g. FGFR2 binds FGF1 to FGF10, whereas FGFR3 binds FGF1/2/4/8/9/17/18), creating a complex signaling network where a single FGF can induce distinct cellular responses. This notion is highlighted in a recent article by Duong et al. (196), in which the authors report FGF8 to function as cell fate switch that controls the differentiation of radial glial cells in the SVZ into neurons or astrocytes. Additional studies have demonstrated that FGFs regulate astrocyte morphogenesis, maturation, and function in both health and disease (197–199). For instance, in remyelinating lesions of MS, FGF-1 may act as a promoter of remyelination by an indirect mechanism that involves the induction of CXCL8 and LIF expression in astrocytes (204).
Furthermore, astrocytes have been recognized as important source of FGFs (192). Indeed, reactive astrocytes have been found to upregulate FGF2 expression following CNS insult in multiple species in vivo (89, 90) and in vitro (205). In particular, a study by Messersmith et al. (90) found significantly increased FGF2 mRNA transcripts and protein levels associated to white matter astrocytes in the initial phase of remyelination, indicating that astrocyte-derived FGF2 may modulate the differentiation of oligodendrocytes (206) (Figure 2). Other potential effects of astrocyte-derived FGF2 include the attenuation of neuronal death via signaling through FGFR3 (207) and autocrine/paracrine regulation of glia reactivity (199). The therapeutic potential of FGF2 is recapitulated in a comprehensive study by Ruffini et al. (208), in which the authors demonstrate that viral delivery of FGF2 to the CNS of mice 1 week after EAE induction significantly ameliorated the clinicopathological outcome, marked by reduced infiltration of peripheral immune cells, and an increase of myelin-forming oligodendrocytes. It is unclear, however, to what extent astrocytes contribute to these beneficial effects of FGF2. Indeed, several reports suggest that FGF2 in general, and astrocyte-derived FGF2 in particular can also inhibit oligodendrocyte repopulation and their remyelinating capacities in multiple models of CNS insult (206, 209–211). Besides FGF2, astrocyte-derived FGF9 has been implicated to play a role during remyelination and CNS inflammation (212). Lindner et al. (212) demonstrated in a series of in vitro experiments and post-mortem tissue analyses of MS patients that FGF9, upregulated by astrocytes following CNS insult, inhibits remyelination and induces the expression of inflammatory genes in oligodendrocytes. Overall, the existing data fails to produce a coherent picture on under which conditions FGF family members exhibit beneficial or harmful functions during CNS insult (206, 211) and extensive research is needed to illuminate the effects of astrocyte-derived FGFs. Nevertheless, accumulating evidence strongly suggests that FGFs play an important role in the pathophysiology of MS and (209, 212, 213) and new insights may guide the development of FGF-based therapeutic strategies.
HB-EGF
HB-EGF has originally been identified in macrophage-like cells with mitogenic functions for numerous cell types (214). Similar to NGF and other neurotrophins, HB-EGF is synthesized in a pre-mature transmembrane form (pro-HB-EGF) before it is cleaved by numerous metalloproteinases (MMP3, MMP9, ADM9, ADAM10, ADAM12, ADAM17) into its mature, soluble form (215). While the membrane anchored pro-HB-EGF functions as juxtacrine growth factor and receptor for diphtheria toxin in some species, soluble HB-EGF has recently been described to modulate cell migration, differentiation, and inflammatory functions in multiple cell types (216–222). In addition, HB-EGF enhances neurogenesis in models of ischemic injury and promotes the survival of dopaminergic neurons (223, 224) (Figure 2). Mature HB-EGF signals through EGFR, ErbB4 and a newly defined N-arginine dibasic convertase, but may also be able to induce ErbB2 through heterodimerization (214, 225–227). In astrocytes, upregulation of HBEGF mRNA has been observed in response to sphingosine-1-phosphat (S1P)-receptor activation by S1P or S1P receptor modulator fingolimod (92, 93). This may be dependent on combined S1P1R and S1P2R signaling and the activation of the immediate early transcription factors ERG1 and AP1, indicating that astrocyte-derived HB-EGF is part of a rapid response mechanism that counteracts pro-inflammatory astrocyte functions (93). Indeed, it has been suggested that HB-EGF suppresses the nuclear translocation of NF-κB by inhibition of IκB kinase (IKK) mediated inhibitor of κB (IκB) degradation (222). Collectively, astrocyte-derived HB-EGF may not only serve as neurotrophic factor but also dampen pro-inflammatory gene transcription in Egfr-expressing microglia and infiltrating immune cells (220).
IGF
Insulin-like growth factor 1 (IGF-1) is a polypeptide hormone and functions as primary mediator of growth hormone (GH) dependent growth effects in most peripheral tissues (228). In the brain, IGF-1 regulates the proliferation and differentiation of multiple CNS resident cells and has been implicated in several neurological disorders (229–231). IGF-1 signals through its cognate receptor IGFR-1R, but can also form functional hybrids with the insulin receptor (229). Besides IGF-1, IGF-2 and its receptor IGF-2R share a similar expression pattern in the developing and mature CNS (229). Both IGF/IGFR pairs signal through phosphoinositide 3-kinase (PI3K)–AKT–forkhead box protein O (FOXO) and RAS–mitogen-activated protein kinase (MAPK) pathways to induce downstream expression of growth promoting genes. Interestingly, IGF-1R can furthermore modulate transcription directly by acting as transcriptional regulator in the nucleus (232). Numerous studies indicate roles for IGF-1/IGF-1R signaling in the pathogenesis and progression of neurological disorders and show that their expression is differentially modulated by CNS insult (159, 229, 231, 233, 234). While microglia have been implicated as main source of IGFs under pathological conditions, the neuroprotective potential of astrocyte-derived IGFs has recently gained attention (94–96). For example, conditional overexpression of IGF-1 in astrocytes promoted neuronal survival and reduced hippocampal neurodegeneration in a controlled cortical injury (CCI) model, highlighting the therapeutic efficacy of astrocyte-derived IGFs (96) (Figure 2). Although the role of endogenous, astrocyte-derived IGFs in the context of neuroinflammation must be further investigated, its broad spectrum of growth promoting effects on CNS-resident cells may provide beneficial for neuronal and non-neuronal regeneration.
TGF-β
Transforming growth factor β (TGF-β) belongs to a family of pleiotrophic cytokines with potent regulatory and inflammatory functions in numerous cell types (235–237). In mammals, TGF-β exists in three isoforms (TGF-β1. TGF-β2, TGF-β3), with TGF-β1 being the most prevalent one. The immunoregulatory cytokine elicits its function through binding to TGF-β type I (TGF-βRI) and type II (TGF-βRII) receptors, which induce Smad protein phosphorylation and downstream transcriptional regulation of their target genes (236, 238). Generally, TGF-β has been identified as master regulator of immune tolerance, T cell differentiation and mediator of inflammatory responses in multiple cell types (235, 236, 239, 240). In addition, work by Krieglstein and others suggests that TGF-β also exerts neurotrophic functions through direct or indirect regulation of neuronal development and survival (143, 241–248) (Figure 2). While members of the TGF-β superfamily are widely expressed among numerous cell types in the CNS, astrocytes have been implicated as key contributor of endogenous TGF-β in the CNS (249). Indeed, astrocyte-derived TGF-β has been linked to anti-inflammatory and neuroprotective functions in models of experimental stroke, Toxoplasma infection, and AD (97, 250, 251). Although the molecular mechanisms underlying the anti-inflammatory and neuroprotective functions of astrocyte-derived TGF-β in the context of neuroinflammation remain to be defined, TGF-β may exert its beneficial role through the suppression of glial NF-κB signaling and the associated pro-inflammatory functions of CNS resident macrophages and microglia (97) (Figure 2). This is in line with a study defining an IL-10/TGF-β signaling loop between activated astrocytes and microglia that limits CNS inflammation (98). Microglia-derived IL-10, an anti-inflammatory cytokine, redirected astrocyte pathogenic functions and stimulated the production of TGF-β, which in turn reduced microglial activation and the secretion of pro-inflammatory IL-1β (98). Taken together, astrocyte-derived TGF-β may serve as immunosuppressive cytokine during initial inflammation while its neurotrophic functions support axonal regeneration during recovery.
LIF
Leukemia inhibitory factor (LIF) is another member of the IL-6 class cytokine family. Analogous to CNTF, LIF signals through LIFRα and gp130 to induce JAK/STAT dependent gene transcription. It was first described as a suppressor of proliferation in a myeloid leukemia cell line, but has since been associated to functions in multiple peripheral organs (252–257). In addition, LIF has been recognized as neuropoetic cytokine, regulating the differentiation and activation of multiple cell types in the CNS (258–262). Under homeostatic conditions, expression of Lif remains low in the CNS, but is heavily ramped up in response to various types of insult (99, 100, 263–265). Astrocytes are thought to play an important role in the upregulation of LIF, and have been identified as major source of Lif mRNA in the injured brain (100, 263). Consequently, astrocyte-derived LIF may potentiate stem cell renewal in the adult SVZ and increase the regenerative capacities following CNS insult (101, 262) (Figure 2). Although it is not entirely clear what mechanisms modulate the upregulation of Lif expression in astrocytes, S1PR signaling has been shown to be a potent inducer (92, 93). Apart from its beneficial functions on stem cell regeneration and neurogenesis, accumulating evidence shows that LIF plays essential roles during oligodendrocyte maturation and function in the context of autoimmune inflammation and remyelination (102–107). This becomes important both in health and disease. A study by Ishibashi (100) demonstrated that astrocytes secrete LIF in response to ATP stimulation and promote the oligodendrocyte-mediated myelination of axons, defining a mechanism that mediates myelination in an activity-dependent manner. During EAE, increased levels of LIF have been associated to protective functions and increased survival of oligodendrocytes (102, 107) (Figure 2). In line with this notion, blockage of LIF worsened oligodendrocyte loss while conditional deletion of a LIFR/gp130 suppressor protected against cuprizone-induced demyelination (102, 266). Aside from oligodendrocytes, LIF has been implicated in the regulation of T-cell responses by altering their pathogenic potential. Indeed, several studies show that LIF suppresses pro-inflammatory gene expression in CD4 T-cells and skews their polarization in an anti-inflammatory manner (267–269) (Figure 2). Collectively, these data suggest that astrocyte-derived LIF may contribute to the resolution of acute tissue inflammation, promote the remyelinating capacities of oligodendrocytes, and induce stem-cell renewal to prevent long-term neurodegeneration.
Non-Secreted Factors
In addition to neurotrophic factors, neuropoetic cytokines and growth factors, astrocytes secrete a plethora of other protective factors, including cytokines, metabolites, extracellular matrix (ECM) proteins, and metalloproteinases (MMPs) (23, 50, 266, 267, 270). In the healthy brain, tight metabolic coupling between neurons and astrocytes is key to sustain high firing rates and neuronal wellbeing (267). Recently, it has been suggested that the metabolic crosstalk between astrocytes and neurons also plays important roles during neuroinflammation and neurodegeneration (271–273). In this context, it remains to be defined which metabolites with protective functions during homeostasis have similar effects in the inflamed or injured brain. Similarly, astrocyte-derived ECM proteins and MMPs have been associated to numerous protective functions in the healthy brain. However, it has been well documented that astrocyte-derived chondroitin sulfate proteoglycans (GSPGs), which are a key component of the ECM, restrict remyelination, neurite outgrowth and limit functional recovery following CNS injury (274). Among multiple other strategies to overcome CSPG mediated inhibition of neuronal regeneration, MMPs have been proposed to exhibit protective effects in the post-acute phase of CNS injury (275, 276). Indeed, astrocyte-derived MMPs may promote neuronal plasticity in the healthy brain and enhance functional recovery through ECM dependent and independent mechanisms (277, 278). Future research will need to determine which parameters dictate the protective effects of astrocyte-derived ECM components and MMPs, and how they can be harnessed for therapeutic strategies to enhance recovery following CNS insult.
Therapeutic Outlook and Discussion
Currently, only few effective therapies exist to tackle the vast complexity of neurological disorders and the development of novel strategies is hampered by their limited access to the CNS. Exogenously administered agents require a high permeability through the BBB and a persisting bioavailability to ensure long-lasting therapeutic effects (279). Only a limited number of small molecules has shown beneficial “protective” effects on glial cells following acute CNS insult so far, which is best documented during neuroinflammation (92, 280–286). Thus, there is a dire need for novel strategies that mediate recovery after acute CNS insult and lead to long-term regeneration in chronic inflammatory and degenerative diseases. Based on their strategic location and versatile roles in the pathogenesis and progression of CNS disorders, astrocytes have been proposed as therapeutic targets (230, 231). Generally, most existing approaches targeting astrocytes in the context of neurological disorders are based on gene therapy, cell replacement, or the exogenous administration of compounds that induce neuroprotective functions in astrocytes (287, 288). As discussed above, multiple exogenously administered molecules mimic the protective functions of astrocyte-derived mediators or induce their endogenous production (70, 73, 84, 92, 93, 96, 148, 149, 160). Although these strategies represent promising approaches, several issues will need to be addressed.
First, the multi-faceted functions of astrocyte derived mediators are determined by their differential spatial and temporal expression (18, 289–293). Consequently, exogenous activation of astrocytes at an improper time-point and in the wrong microenvironment might result in harmful, rather than beneficial effects, ultimately worsening clinical outcome. Further research is needed to determine (1) how many functionally distinct astrocyte-subsets exist, (2) which factors induce their differentiation, (3) how the underlying transcriptional programs relate to the differential secretion of astrocyte-derived mediators, and (4) whether these transcriptional subsets also correlate with different secretional and functional astrocyte subpopulations.
Most protective effects mediated by astrocytes are the result of a transient response to environmental cues present in the disease-specific micro-environment. It is conceivable that the diversity and strength of the intercellular crosstalk, specific to a given lesion type, also strongly influences the outcome of specific therapeutic strategies. Indeed, while a transient and highly disease-specific astrocyte response allows for an adapted reaction to the respective insult and prevents extensive overactivation/-suppression, exogenous induction of specific tissue-protective pathways may only provide short-term solutions to long-term problems, and eventually wear off when the local microenvironment changes over the course of the disease.
In these lines, genetic modifications of astrocytes to foster their tissue-protective and anti-inflammatory functions have been proposed (70, 73, 84, 96, 148, 149, 288). These approaches might be particularly useful for the treatment of chronic conditions and allow for the targeted activation of protective subpopulations. In this context, adeno-associated viruses (AAVs) have been proven to be efficient vectors for viral gene delivery. Interestingly, a landmark study by Foust and colleagues (294) demonstrated that AAV serotype 9 successfully bypasses the BBB and predominantly transduces astrocytes in the adult mouse brain (295). Further modifications such as the conditional expression of target genes under the astrocyte-specific GFAP promoter may enhance viral delivery and present a robust delivery system (296, 297). However, AAVs are limited to a relatively small insert size (4.7 kb) and viral delivery may have unpredictable off-target effects. To overcome this complication, cell replacement strategies (using genetically modified or unmodified cells) may present a useful alternative to harness the anti-inflammatory and tissue-protective functions of astrocytes. Several transplantation trials of human fetal mesencephalic stem cells into striatal regions of PD patients have demonstrated successful functional integration and long-term benefits (298–302). This is different for astrocytes, as they require differentiation into their mature form before being grafted. Although we have little information about whether human astrocytes can be generated from embryonic stem cells (ESCs) or NSCs, several studies report successful and stable differentiation of human ESCs into dopaminergic neurons, and transplantation of glial-restricted pluripotent stem cells in mouse models of ALS suggest that this might represent a feasible approach (303–307). One significant advantage over existing cell replacement therapies using neurons is that one astrocyte has the capacity to induce differentiation and survival in numerous neurons (through the secretion of soluble factors), thus making it an efficient approach to tackle neurodegeneration. Indeed, two ongoing Phase I/IIa trials (NCT03482050, with GDNF overexpression NCT02943850) currently examine the therapeutic potential of grafted human stem cell derived astrocytes for the treatment of ALS.
Overall, numerous studies presented in this review suggest that exogenous application or the genetic overexpression of astrocyte derived factors limit inflammation and aid central nervous regeneration. These findings need to be strengthened and extensively recapitulated in other clinically relevant model species and CNS injuries before taking the next step towards clinical application. Such models will allow to find common mechanisms underlying tissue-protective functions of astrocytes and assess their translatability in a defined setting. Furthermore, it will become essential to investigate the combinatorial effects of astrocyte-derived factors as multiple studies have demonstrated synergistic effects and cross-regulatory mechanisms between several of the discussed mediators (308–315). Lastly, we are just beginning to grasp the versatile roles glial cells play in the diseased CNS and the extensive characterization of astrocytic subsets beyond a dualistic concept will be inevitable to understand their roles in the context of CNS inflammation. Novel high-throughput technologies will pave the way for a better understanding of what signals drive the secretion of protective factors by astrocytes, to what extent these transcriptional profiles are influenced by intercellular communication, and how we can harness the protective potential of reactive astrocytes in a clinical setting.
Author Contributions
Both ML and VR researched data and reviewed and edited the manuscript. ML wrote the manuscript.
Funding
This work was supported by grants RO4866/2-1 and RO4866/4-1 from the German Research foundation (DFG) and an ERC Starting Grant (851693 – HICI) by the European Research Council.
Conflict of Interest
The authors declare that the research was conducted in the absence of any commercial or financial relationships that could be construed as a potential conflict of interest.
References
1. Molofsky AV, Deneen B. Astrocyte development: A Guide for the Perplexed. Glia (2015) 63(8):1320–9. doi: 10.1002/glia.22836
2. Hochstim C, Deneen B, Lukaszewicz A, Zhou Q, Anderson DJ. Identification of positionally distinct astrocyte subtypes whose identities are specified by a homeodomain code. Cell (2008) 133(3):510–22. doi: 10.1016/j.cell.2008.02.046
3. Molofsky AV, Kelley KW, Tsai H-H, Redmond SA, Chang SM, Madireddy L, et al. Astrocyte-encoded positional cues maintain sensorimotor circuit integrity. Nature (2014) 509(7499):189–94. doi: 10.1038/nature13161
4. Ge W-P, Miyawaki A, Gage FH, Jan YN, Jan LY. Local generation of glia is a major astrocyte source in postnatal cortex. Nature (2012) 484(7394):376–80. doi: 10.1038/nature10959
5. Deneen B, Ho R, Lukaszewicz A, Hochstim CJ, Gronostajski RM, Anderson DJ. The transcription factor NFIA controls the onset of gliogenesis in the developing spinal cord. Neuron (2006) 52(6):953–68. doi: 10.1016/j.neuron.2006.11.019
6. Rowitch DH, Kriegstein AR. Developmental genetics of vertebrate glial–cell specification. Nature (2010) 468(7321):214–22. doi: 10.1038/nature09611
7. Christopherson KS, Ullian EM, Stokes CCA, Mullowney CE, Hell JW, Agah A, et al. Thrombospondins are astrocyte-secreted proteins that promote CNS synaptogenesis. Cell (2005) 120(3):421–33. doi: 10.1016/j.cell.2004.12.020
8. Mauch DH, Nägler K, Schumacher S, Göritz C, Müller E-C, Otto A, et al. CNS Synaptogenesis Promoted by Glia-Derived Cholesterol. Science (2001) 294(5545):1354–7. doi: 10.1126/science.294.5545.1354
9. Hughes EG, Elmariah SB, Balice-Gordon RJ. Astrocyte secreted proteins selectively increase hippocampal GABAergic axon length, branching, and synaptogenesis. Mol Cell Neurosci (2010) 43(1):136–45. doi: 10.1016/j.mcn.2009.10.004
10. Traiffort E, Kassoussi A, Zahaf A, Laouarem Y. Astrocytes and Microglia as Major Players of Myelin Production in Normal and Pathological Conditions. Front Cell Neurosci (2020) 14:79. doi: 10.3389/fncel.2020.00079
11. Hama H, Hara C, Yamaguchi K, Miyawaki A. PKC signaling mediates global enhancement of excitatory synaptogenesis in neurons triggered by local contact with astrocytes. Neuron (2004) 41(3):405–15. doi: 10.1016/S0896-6273(04)00007-8
12. Barker AJ, Koch SM, Reed J, Barres BA, Ullian EM. Developmental control of synaptic receptivity. J Neurosci Off J Soc Neurosci (2008) 28(33):8150–60. doi: 10.1523/JNEUROSCI.1744-08.2008
13. Murai KK, Nguyen LN, Irie F, Yamaguchi Y, Pasquale EB. Control of hippocampal dendritic spine morphology through ephrin-A3/EphA4 signaling. Nat Neurosci (2003) 6(2):153–60. doi: 10.1038/nn994
14. Magistretti PJ. Neuron–glia metabolic coupling and plasticity. J Exp Biol (2006) 209(12):2304–11. doi: 10.1242/jeb.02208
15. Magistretti PJ, Allaman I. Lactate in the brain: from metabolic end-product to signalling molecule. Nat Rev Neurosci (2018) 19(4):235–49. doi: 10.1038/nrn.2018.19
16. Santello M, Toni N, Volterra A. Astrocyte function from information processing to cognition and cognitive impairment. Nat Neurosci (2019) 22(2):154–66. doi: 10.1038/s41593-018-0325-8
17. Pascual O, Casper KB, Kubera C, Zhang J, Revilla-Sanchez R, Sul J-Y, et al. Astrocytic Purinergic Signaling Coordinates Synaptic Networks. Science (2005) 310(5745):113–6. doi: 10.1126/science.1116916
18. Haim LB, Rowitch DH. Functional diversity of astrocytes in neural circuit regulation. Nat Rev Neurosci (2017) 18(1):31–41. doi: 10.1038/nrn.2016.159
19. Santello M, Volterra A. Synaptic modulation by astrocytes via Ca2+-dependent glutamate release. Neuroscience (2009) 158(1):253–9. doi: 10.1016/j.neuroscience.2008.03.039
20. Walz W. Role of astrocytes in the clearance of excess extracellular potassium. Neurochem Int (2000) 36(4):291–300. doi: 10.1016/S0197-0186(99)00137-0
21. Cui Y, Yang Y, Ni Z, Dong Y, Cai G, Foncelle A, et al. Astroglial Kir4.1 in the lateral habenula drives neuronal bursts in depression. Nature (2018) 554(7692):323–7. doi: 10.1038/nature25752
22. Tong X, Ao Y, Faas GC, Nwaobi SE, Xu J, Haustein MD, et al. Astrocyte Kir4.1 ion channel deficits contribute to neuronal dysfunction in Huntington’s disease model mice. Nat Neurosci (2014) 17(5):694–703. doi: 10.1038/nn.3691
23. Song F, Hong X, Cao J, Ma G, Han Y, Cepeda C, et al. Kir4.1 channels in NG2-glia play a role in development, potassium signaling, and ischemia-related myelin loss. Commun Biol (2018) 1(1):1–9. doi: 10.1038/s42003-018-0083-x
24. Obermeier B, Daneman R, Ransohoff RM. Development, maintenance and disruption of the blood-brain barrier. Nat Med (2013) 19(12):1584–96. doi: 10.1038/nm.3407
25. Abbott NJ, Rönnbäck L, Hansson E. Astrocyte–endothelial interactions at the blood–brain barrier. Nat Rev Neurosci (2006) 7(1):41–53. doi: 10.1038/nrn1824
26. Lee S-W, Kim WJ, Choi YK, Song HS, Son MJ, Gelman IH, et al. SSeCKS regulates angiogenesis and tight junction formation in blood-brain barrier. Nat Med (2003) 9(7):900–6. doi: 10.1038/nm889
27. Louveau A, Harris TH, Kipnis J. Revisiting the concept of CNS immune privilege. Trends Immunol (2015) 36(10):569–77. doi: 10.1016/j.it.2015.08.006
28. Sofroniew MV. Astrocyte barriers to neurotoxic inflammation. Nat Rev Neurosci (2015) 16(5):249–63. doi: 10.1038/nrn3898
29. Kipnis J. Multifaceted interactions between adaptive immunity and the central nervous system. Science (2016) 353(6301):766–71. doi: 10.1126/science.aag2638
30. Amiry-Moghaddam M, Ottersen OP. The molecular basis of water transport in the brain. Nat Rev Neurosci (2003) 4(12):991–1001. doi: 10.1038/nrn1252
31. Chung SW, Kim J-Y, Yoon JP, Suh DW, Yeo WJ, Lee Y-S. Atrogin1-induced loss of aquaporin 4 in myocytes leads to skeletal muscle atrophy. Sci Rep (2020) 10(1):14189. doi: 10.1038/s41598-020-71167-8
32. Manley GT, Fujimura M, Ma T, Noshita N, Filiz F, Bollen AW, et al. Aquaporin-4 deletion in mice reduces brain edema after acute water intoxication and ischemic stroke. Nat Med (2000) 6(2):159–63. doi: 10.1038/72256
33. Prydz A, Stahl K, Puchades M, Davarpaneh N, Nadeem M, Ottersen OP, et al. Subcellular expression of aquaporin-4 in substantia nigra of normal and MPTP-treated mice. Neuroscience (2017) 359:258–66. doi: 10.1016/j.neuroscience.2017.07.029
34. Sun H, Liang R, Yang B, Zhou Y, Liu M, Fang F, et al. Aquaporin-4 mediates communication between astrocyte and microglia: Implications of neuroinflammation in experimental Parkinson’s disease. Neuroscience (2016) 317:65–75. doi: 10.1016/j.neuroscience.2016.01.003
35. Jarius S, Paul F, Franciotta D, Waters P, Zipp F, Hohlfeld R, et al. Mechanisms of Disease: aquaporin-4 antibodies in neuromyelitis optica. Nat Clin Pract Neurol (2008) 4(4):202–14. doi: 10.1038/ncpneuro0764
36. Sofroniew MV. Astrogliosis. Cold Spring Harb Perspect Biol (2015) 7(2):a020420. doi: 10.1101/cshperspect.a020420
37. Sofroniew MV. Molecular dissection of reactive astrogliosis and glial scar formation. Trends Neurosci (2009) 32(12):638–47. doi: 10.1016/j.tins.2009.08.002
38. Burda JE, Sofroniew MV. Reactive gliosis and the multicellular response to CNS damage and disease. Neuron (2014) 81(2):229–48. doi: 10.1016/j.neuron.2013.12.034
39. Wheeler MA, Quintana FJ. Regulation of Astrocyte Functions in Multiple Sclerosis. Cold Spring Harb Perspect Med (2019) 02:9(1). doi: 10.1101/cshperspect.a029009
40. Solano RM, Casarejos MJ, Menéndez-Cuervo J, Rodriguez-Navarro JA, García de Yébenes J, Mena MA. Glial Dysfunction in Parkin Null Mice: Effects of Aging. J Neurosci (2008) 28(3):598–611. doi: 10.1523/JNEUROSCI.4609-07.2008
41. Gu X-L, Long C-X, Sun L, Xie C, Lin X, Cai H. Astrocytic expression of Parkinson’s disease-related A53T alpha-synuclein causes neurodegeneration in mice. Mol Brain (2010) 3:12. doi: 10.1186/1756-6606-3-12
42. Anderson MA, Burda JE, Ren Y, Ao Y, O’Shea TM, Kawaguchi R, et al. Astrocyte scar formation aids central nervous system axon regeneration. Nature (2016) 532(7598):195–200. doi: 10.1038/nature17623
43. Adams KL, Gallo V. The diversity and disparity of the glial scar. Nat Neurosci (2018) 21(1):9–15. doi: 10.1038/s41593-017-0033-9
44. Zamanian JL, Xu L, Foo LC, Nouri N, Zhou L, Giffard RG, et al. Genomic analysis of reactive astrogliosis. J Neurosci Off J Soc Neurosci (2012) 32(18):6391–410. doi: 10.1523/JNEUROSCI.6221-11.2012
45. Liddelow SA, Guttenplan KA, Clarke LE, Bennett FC, Bohlen CJ, Schirmer L, et al. Neurotoxic reactive astrocytes are induced by activated microglia. Nature (2017) 541(7638):481–7. doi: 10.1038/nature21029
46. Hamby ME, Coppola G, Ao Y, Geschwind DH, Khakh BS, Sofroniew MV. Inflammatory mediators alter the astrocyte transcriptome and calcium signaling elicited by multiple G-protein-coupled receptors. J Neurosci Off J Soc Neurosci (2012) 32(42):14489–510. doi: 10.1523/JNEUROSCI.1256-12.2012
47. John GR, Lee SC, Song X, Rivieccio M, Brosnan CF. IL-1-regulated responses in astrocytes: relevance to injury and recovery. Glia (2005) 49(2):161–76. doi: 10.1002/glia.20109
48. Rothhammer V, Borucki DM, Tjon EC, Takenaka MC, Chao C-C, Ardura-Fabregat A, et al. Microglial control of astrocytes in response to microbial metabolites. Nature (2018) 557(7707):724–8. doi: 10.1038/s41586-018-0119-x
49. Linnerbauer M, Wheeler MA, Quintana FJ. Astrocyte Crosstalk in CNS Inflammation. Neuron (2020) 0(0). doi: 10.1016/j.neuron.2020.08.012
50. Owens T, Bechmann I, Engelhardt B. Perivascular Spaces and the Two Steps to Neuroinflammation. J Neuropathol Exp Neurol (2008) 67(12):1113–21. doi: 10.1097/NEN.0b013e31818f9ca8
51. Rostami J, Fotaki G, Sirois J, Mzezewa R, Bergström J, Essand M, et al. Astrocytes have the capacity to act as antigen-presenting cells in the Parkinson’s disease brain. J Neuroinflam (2020) 17(1):1–18. doi: 10.1186/s12974-020-01776-7
52. Cornet A, Bettelli E, Oukka M, Cambouris C, Avellana-Adalid V, Kosmatopoulos K, et al. Role of astrocytes in antigen presentation and naive T-cell activation. J Neuroimmunol (2000) 106(1):69–77. doi: 10.1016/S0165-5728(99)00215-5
53. Hamo L, Stohlman SA, Otto-Duessel M, Bergmann CC. Distinct regulation of MHC molecule expression on astrocytes and microglia during viral encephalomyelitis. Glia (2007) 55(11):1169–77. doi: 10.1002/glia.20538
54. Choi C, Park JY, Lee J, Lim J-H, Shin E-C, Ahn YS, et al. Fas Ligand and Fas Are Expressed Constitutively in Human Astrocytes and the Expression Increases with IL-1, IL-6, TNF-α, or IFN-γ. J Immunol (1999) 162(4):1889–95.
55. Krumbholz M, Theil D, Derfuss T, Rosenwald A, Schrader F, Monoranu C-M, et al. BAFF is produced by astrocytes and up-regulated in multiple sclerosis lesions and primary central nervous system lymphoma. J Exp Med (2005) 201(2):195–200. doi: 10.1084/jem.20041674
56. Wang X, Haroon F, Karray S, Deckert M, Schlüter D. Astrocytic Fas ligand expression is required to induce T-cell apoptosis and recovery from experimental autoimmune encephalomyelitis. Eur J Immunol (2013) 43(1):115–24. doi: 10.1002/eji.201242679
57. Baert L, Benkhoucha M, Popa N, Ahmed MC, Manfroi B, Boutonnat J, et al. A proliferation-inducing ligand–mediated anti-inflammatory response of astrocytes in multiple sclerosis. Ann Neurol (2019) 85(3):406–20. doi: 10.1002/ana.25415
58. Farina C, Aloisi F, Meinl E. Astrocytes are active players in cerebral innate immunity. Trends Immunol (2007) 28(3):138–45. doi: 10.1016/j.it.2007.01.005
59. Messing A, Brenner M. GFAP: Functional implications gleaned from studies of genetically engineered mice. Glia (2003) 43(1):87–90. doi: 10.1002/glia.10219
60. Mayo L, Trauger SA, Blain M, Nadeau M, Patel B, Alvarez JI, et al. Regulation of astrocyte activation by glycolipids drives chronic CNS inflammation. Nat Med (2014) 20(10):1147–56. doi: 10.1038/nm.3681
61. Toft-Hansen H, Füchtbauer L, Owens T. Inhibition of reactive astrocytosis in established experimental autoimmune encephalomyelitis favors infiltration by myeloid cells over T cells and enhances severity of disease. Glia (2011) 59(1):166–76. doi: 10.1002/glia.21088
62. Voskuhl RR, Peterson RS, Song B, Ao Y, Morales LBJ, Tiwari-Woodruff S, et al. Reactive Astrocytes Form Scar-Like Perivascular Barriers to Leukocytes during Adaptive Immune Inflammation of the CNS. J Neurosci (2009) 29(37):11511–22. doi: 10.1523/JNEUROSCI.1514-09.2009
63. Colombo E, Farina C. Astrocytes: Key Regulators of Neuroinflammation. Trends Immunol (2016) 37(9):608–20. doi: 10.1016/j.it.2016.06.006
64. Rothhammer V, Quintana FJ. Control of autoimmune CNS inflammation by astrocytes. Semin Immunopathol (2015) 37(6):625–38. doi: 10.1007/s00281-015-0515-3
65. Bush TG, Puvanachandra N, Horner CH, Polito A, Ostenfeld T, Svendsen CN, et al. Leukocyte Infiltration, Neuronal Degeneration, and Neurite Outgrowth after Ablation of Scar-Forming, Reactive Astrocytes in Adult Transgenic Mice. Neuron (1999) 23(2):297–308. doi: 10.1016/S0896-6273(00)80781-3
66. Faulkner JR, Herrmann JE, Woo MJ, Tansey KE, Doan NB, Sofroniew MV. Reactive astrocytes protect tissue and preserve function after spinal cord injury. J Neurosci Off J Soc Neurosci (2004) 24(9):2143–55. doi: 10.1523/JNEUROSCI.3547-03.2004
67. Li L, Lundkvist A, Andersson D, Wilhelmsson U, Nagai N, Pardo AC, et al. Protective role of reactive astrocytes in brain ischemia. J Cereb Blood Flow Metab Off J Int Soc Cereb Blood Flow Metab (2008) 28(3):468–81. doi: 10.1038/sj.jcbfm.9600546
68. Fulmer CG, VonDran MW, Stillman AA, Huang Y, Hempstead BL, Dreyfus CF. Astrocyte-derived BDNF supports myelin protein synthesis after cuprizone-induced demyelination. J Neurosci Off J Soc Neurosci (2014) 34(24):8186–96. doi: 10.1523/JNEUROSCI.4267-13.2014
69. Linker RA, Lee D-H, Demir S, Wiese S, Kruse N, Siglienti I, et al. Functional role of brain-derived neurotrophic factor in neuroprotective autoimmunity: therapeutic implications in a model of multiple sclerosis. Brain (2010) 133(8):2248–63. doi: 10.1093/brain/awq179
70. Quesseveur G, David DJ, Gaillard MC, Pla P, Wu MV, Nguyen HT, et al. BDNF overexpression in mouse hippocampal astrocytes promotes local neurogenesis and elicits anxiolytic-like activities. Transl Psychiatry (2013) 3(4):e253–3. doi: 10.1038/tp.2013.30
71. Domeniconi M, Hempstead BL, Chao MV. Pro-NGF secreted by astrocytes promotes motor neuron cell death. Mol Cell Neurosci (2007) 34(2):271–9. doi: 10.1016/j.mcn.2006.11.005
72. Cheng Y-Y, Zhao H-K, Chen L-W, Yao X-Y, Wang Y-L, Huang Z-W, et al. Reactive astrocytes increase expression of proNGF in the mouse model of contused spinal cord injury. Neurosci Res (2019) 157:34–43. doi: 10.1016/j.neures.2019.07.007
73. Villoslada P, Hauser SL, Bartke I, Unger J, Heald N, Rosenberg D, et al. Human nerve growth factor protects common marmosets against autoimmune encephalomyelitis by switching the balance of T helper cell type 1 and 2 cytokines within the central nervous system. J Exp Med (2000) 191(10):1799–806. doi: 10.1084/jem.191.10.1799
74. Rizzi C, Tiberi A, Giustizieri M, Marrone MC, Gobbo F, Carucci NM, et al. NGF steers microglia toward a neuroprotective phenotype. Glia (2018) 66(7):1395–416. doi: 10.1002/glia.23312
75. Kostianovsky AM, Maier LM, Anderson RC, Bruce JN, Anderson DE. Astrocytic Regulation of Human Monocytic/Microglial Activation. J Immunol (2008) 181(8):5425–32. doi: 10.4049/jimmunol.181.8.5425
76. Rocha SM, Cristovão AC, Campos FL, Fonseca CP, Baltazar G. Astrocyte-derived GDNF is a potent inhibitor of microglial activation. Neurobiol Dis (2012) 47(3):407–15. doi: 10.1016/j.nbd.2012.04.014
77. Drinkut A, Tereshchenko Y, Schulz JB, Bähr M, Kügler S. Efficient Gene Therapy for Parkinson’s Disease Using Astrocytes as Hosts for Localized Neurotrophic Factor Delivery. Mol Ther (2012) 20(3):534–43. doi: 10.1038/mt.2011.249
78. Sandhu JK, Gardaneh M, Iwasiow R, Lanthier P, Gangaraju S, Ribecco-Lutkiewicz M, et al. Astrocyte-secreted GDNF and glutathione antioxidant system protect neurons against 6OHDA cytotoxicity. Neurobiol Dis (2009) 33(3):405–14. doi: 10.1016/j.nbd.2008.11.016
79. Igarashi Y, Utsumi H, Chiba H, Yamada-Sasamori Y, Tobioka H, Kamimura Y, et al. Glial cell line-derived neurotrophic factor induces barrier function of endothelial cells forming the blood-brain barrier. Biochem Biophys Res Commun (1999) 261(1):108–12. doi: 10.1006/bbrc.1999.0992
80. Appel E, Kolman O, Kazimirsky G, Blumberg PM, Brodie C. Regulation of GDNF expression in cultured astrocytes by inflammatory stimuli. NeuroReport (1997) 8(15):3309–12. doi: 10.1097/00001756-199710200-00023
81. Keasey MP, Kang SS, Lovins C, Hagg T. Inhibition of a novel specific neuroglial integrin signaling pathway increases STAT3-mediated CNTF expression. Cell Commun Signal CCS (2013) 11:35. doi: 10.1186/1478-811X-11-35
82. Kang SS, Keasey MP, Cai J, Hagg T. Loss of neuron-astroglial interaction rapidly induces protective CNTF expression after stroke in mice. J Neurosci Off J Soc Neurosci (2012) 32(27):9277–87. doi: 10.1523/JNEUROSCI.1746-12.2012
83. Linker RA, Mäurer M, Gaupp S, Martini R, Holtmann B, Giess R, et al. CNTF is a major protective factor in demyelinating CNS disease: A neurotrophic cytokine as modulator in neuroinflammation. Nat Med (2002) 8(6):620–4. doi: 10.1038/nm0602-620
84. Cheng L, Zhao H, Zhang W, Liu B, Liu Y, Guo Y, et al. Overexpression of conserved dopamine neurotrophic factor (CDNF) in astrocytes alleviates endoplasmic reticulum stress-induced cell damage and inflammatory cytokine secretion. Biochem Biophys Res Commun (2013) 435(1):34–9. doi: 10.1016/j.bbrc.2013.04.029
85. Shen Y, Sun A, Wang Y, Cha D, Wang H, Wang F, et al. Upregulation of mesencephalic astrocyte-derived neurotrophic factor in glial cells is associated with ischemia-induced glial activation. J Neuroinflam (2012) 9(1):1–13. doi: 10.1186/1742-2094-9-254
86. Zhao H, Liu Y, Cheng L, Liu B, Zhang W, Guo Y-J, et al. Mesencephalic astrocyte-derived neurotrophic factor inhibits oxygen-glucose deprivation-induced cell damage and inflammation by suppressing endoplasmic reticulum stress in rat primary astrocytes. J Mol Neurosci MN (2013) 51(3):671–8. doi: 10.1007/s12031-013-0042-4
87. Vana AC, Flint NC, Harwood NE, Le TQ, Fruttiger M, Armstrong RC. Platelet-derived growth factor promotes repair of chronically demyelinated white matter. J Neuropathol Exp Neurol (2007) 66(11):975–88. doi: 10.1097/NEN.0b013e3181587d46
88. Woodruff RH, Fruttiger M, Richardson WD, Franklin RJM. Platelet-derived growth factor regulates oligodendrocyte progenitor numbers in adult CNS and their response following CNS demyelination. Mol Cell Neurosci (2004) 25(2):252–62. doi: 10.1016/j.mcn.2003.10.014
89. Fahmy GH, Moftah MZ. FGF-2 in Astroglial Cells During Vertebrate Spinal Cord Recovery. Front Cell Neurosci (2010) 4:129. doi: 10.3389/fncel.2010.00129
90. Messersmith DJ, Murtie JC, Le TQ, Frost EE, Armstrong RC. Fibroblast growth factor 2 (FGF2) and FGF receptor expression in an experimental demyelinating disease with extensive remyelination. J Neurosci Res (2000) 62(2):241–56. doi: 10.1002/1097-4547(20001015)62:2<241::AID-JNR9>3.0.CO;2-D
91. Bethel A, Kirsch JR, Koehler RC, Finklestein SP, Traystman RJ. Intravenous basic fibroblast growth factor decreases brain injury resulting from focal ischemia in cats. Stroke (1997) 28(3):609–615; discussion 615-616. doi: 10.1161/01.STR.28.3.609
92. Hoffmann FS, Hofereiter J, Rübsamen H, Melms J, Schwarz S, Faber H, et al. Fingolimod induces neuroprotective factors in human astrocytes. J Neuroinflam (2015) 12:184. doi: 10.1186/s12974-015-0393-6
93. Tran C, Heng B, Teo JD, Humphrey SJ, Qi Y, Couttas TA, et al. Sphingosine 1-phosphate but not Fingolimod protects neurons against excitotoxic cell death by inducing neurotrophic gene expression in astrocytes. J Neurochem (2020) 153(2):e14917. doi: 10.1111/jnc.14917
94. Chen W, He B, Tong W, Zeng J, Zheng P. Astrocytic Insulin-Like Growth Factor-1 Protects Neurons Against Excitotoxicity. Front Cell Neurosci (2019) 13:298. doi: 10.3389/fncel.2019.00298
95. Pitt J, Wilcox KC, Tortelli V, Diniz LP, Oliveira MS, Dobbins C, et al. Neuroprotective astrocyte-derived insulin/insulin-like growth factor 1 stimulates endocytic processing and extracellular release of neuron-bound Aβ oligomers. Mol Biol Cell (2017) 28(20):2623–36. doi: 10.1091/mbc.e17-06-0416
96. Madathil SK, Carlson SW, Brelsfoard JM, Ye P, D’Ercole AJ, Saatman KE. Astrocyte-Specific Overexpression of Insulin-Like Growth Factor-1 Protects Hippocampal Neurons and Reduces Behavioral Deficits following Traumatic Brain Injury in Mice. PloS One (2013) 8(6):e67204. doi: 10.1371/journal.pone.0067204
97. Cekanaviciute E, Dietrich HK, Axtell RC, Williams AM, Egusquiza R, Wai KM, et al. Astrocytic TGF-β signaling limits inflammation and reduces neuronal damage during central nervous system Toxoplasma infection. J Immunol Baltim Md (1950) 2014193(1):139–49. doi: 10.4049/jimmunol.1303284
98. Norden DM, Fenn AM, Dugan A, Godbout JP. TGFβ produced by IL-10 redirected astrocytes attenuates microglial activation. Glia (2014) 62(6):881–95. doi: 10.1002/glia.22647
99. Bauer S, Rasika S, Han J, Mauduit C, Raccurt M, Morel G, et al. Leukemia inhibitory factor is a key signal for injury-induced neurogenesis in the adult mouse olfactory epithelium. J Neurosci Off J Soc Neurosci (2003) 23(5):1792–803. doi: 10.1523/JNEUROSCI.23-05-01792.2003
100. Ishibashi T, Dakin KA, Stevens B, Lee PR, Kozlov SV, Stewart CL, et al. Astrocytes promote myelination in response to electrical impulses. Neuron (2006) 49(6):823–32. doi: 10.1016/j.neuron.2006.02.006
101. Suzuki S, Yamashita T, Tanaka K, Hattori H, Sawamoto K, Okano H, et al. Activation of cytokine signaling through leukemia inhibitory factor receptor (LIFR)/gp130 attenuates ischemic brain injury in rats. J Cereb Blood Flow Metab Off J Int Soc Cereb Blood Flow Metab (2005) 25(6):685–93. doi: 10.1038/sj.jcbfm.9600061
102. Butzkueven H, Emery B, Cipriani T, Marriott MP, Kilpatrick TJ. Endogenous leukemia inhibitory factor production limits autoimmune demyelination and oligodendrocyte loss. Glia (2006) 53(7):696–703. doi: 10.1002/glia.20321
103. Rittchen S, Boyd A, Burns A, Park J, Fahmy TM, Metcalfe S, et al. Myelin repair in vivo is increased by targeting oligodendrocyte precursor cells with nanoparticles encapsulating leukaemia inhibitory factor (LIF). Biomaterials (2015) 56:78–85. doi: 10.1016/j.biomaterials.2015.03.044
104. Levy YA, Mausner-Fainberg K, Vaknin-Dembinsky A, Amidror T, Regev K, Karni A. Dysregulated production of leukemia inhibitory factor in immune cells of relapsing remitting multiple sclerosis patients. J Neuroimmunol (2015) 278:85–9. doi: 10.1016/j.jneuroim.2014.12.010
105. Kerr BJ, Patterson PH. Leukemia inhibitory factor promotes oligodendrocyte survival after spinal cord injury. Glia (2005) 51(1):73–9. doi: 10.1002/glia.20177
106. Marriott MP, Emery B, Cate HS, Binder MD, Kemper D, Wu Q, et al. Leukemia inhibitory factor signaling modulates both central nervous system demyelination and myelin repair. Glia (2008) 56(6):686–98. doi: 10.1002/glia.20646
107. Butzkueven H, Zhang J-G, Soilu-Hanninen M, Hochrein H, Chionh F, Shipham KA, et al. LIF receptor signaling limits immune-mediated demyelination by enhancing oligodendrocyte survival. Nat Med (2002) 8(6):613–9. doi: 10.1038/nm0602-613
108. Barres BA, Schmid R, Sendnter M, Raff MC. Multiple extracellular signals are required for long-term oligodendrocyte survival. Dev Camb Engl (1993) 118(1):283–95.
109. Zhang Y, Chen K, Sloan SA, Bennett ML, Scholze AR, O’Keeffe S, et al. An RNA-Sequencing Transcriptome and Splicing Database of Glia, Neurons, and Vascular Cells of the Cerebral Cortex. J Neurosci (2014) 34(36):11929–47. doi: 10.1523/JNEUROSCI.1860-14.2014
110. Zhang Y, Sloan SA, Clarke LE, Caneda C, Plaza CA, Blumenthal PD, et al. Purification and Characterization of Progenitor and Mature Human Astrocytes Reveals Transcriptional and Functional Differences with Mouse. Neuron (2016) 89(1):37–53. doi: 10.1016/j.neuron.2015.11.013
111. Magdaleno S, Jensen P, Brumwell CL, Seal A, Lehman K, Asbury A, et al. BGEM: an in situ hybridization database of gene expression in the embryonic and adult mouse nervous system. PloS Biol (2006) 4(4):e86. doi: 10.1371/journal.pbio.0040086
112. Thompson CL, Ng L, Menon V, Martinez S, Lee C-K, Glattfelder K, et al. A high-resolution spatiotemporal atlas of gene expression of the developing mouse brain. Neuron (2014) 83(2):309–23. doi: 10.1016/j.neuron.2014.05.033
113. Wu H, Friedman WJ, Dreyfus CF. Differential regulation of neurotrophin expression in basal forebrain astrocytes by neuronal signals. J Neurosci Res (2004) 76(1):76–85. doi: 10.1002/jnr.20060
114. Dougherty KD, Dreyfus CF, Black IB. Brain-derived neurotrophic factor in astrocytes, oligodendrocytes, and microglia/macrophages after spinal cord injury. Neurobiol Dis (2000) 7(6 Pt B):574–85. doi: 10.1006/nbdi.2000.0318
115. Tokumine J, Kakinohana O, Cizkova D, Smith DW, Marsala M. Changes in spinal GDNF, BDNF, and NT-3 expression after transient spinal cord ischemia in the rat. J Neurosci Res (2003) 74(4):552–61. doi: 10.1002/jnr.10760
116. Cragnolini AB, Friedman WJ. The function of p75NTR in glia. Trends Neurosci (2008) 31(2):99–104. doi: 10.1016/j.tins.2007.11.005
117. Arévalo JC, Wu SH. Neurotrophin signaling: many exciting surprises! Cell Mol Life Sci CMLS (2006) 63(13):1523–37. doi: 10.1007/s00018-006-6010-1
118. Lee R, Kermani P, Teng KK, Hempstead BL. Regulation of Cell Survival by Secreted Proneurotrophins. Science (2001) 294(5548):1945–8. doi: 10.1126/science.1065057
119. Gravel C, Götz R, Lorrain A, Sendtner M. Adenoviral gene transfer of ciliary neurotrophic factor and brain-derived neurotrophic factor leads to long-term survival of axotomized motor neurons. Nat Med (1997) 3(7):765–70. doi: 10.1038/nm0797-765
120. McTigue DM, Horner PJ, Stokes BT, Gage FH. Neurotrophin-3 and Brain-Derived Neurotrophic Factor Induce Oligodendrocyte Proliferation and Myelination of Regenerating Axons in the Contused Adult Rat Spinal Cord. J Neurosci (1998) 18(14):5354–65. doi: 10.1523/JNEUROSCI.18-14-05354.1998
121. Lee D-H, Geyer E, Flach A-C, Jung K, Gold R, Flügel A, et al. Central nervous system rather than immune cell-derived BDNF mediates axonal protective effects early in autoimmune demyelination. Acta Neuropathol (Berl) (2012) 123(2):247–58. doi: 10.1007/s00401-011-0890-3
122. Lindquist S, Schott BH, Ban M, Compston DAS, Sawcer S, Sailer M. The BDNF-Val66Met polymorphism: Implications for susceptibility to multiple sclerosis and severity of disease. J Neuroimmunol (2005) 167(1):183–5. doi: 10.1016/j.jneuroim.2005.06.008
123. Zivadinov R, Weinstock-Guttman B, Benedict R, Tamaño-Blanco M, Hussein S, Abdelrahman N, et al. Preservation of gray matter volume in multiple sclerosis patients with the Met allele of the rs6265 (Val66Met) SNP of brain-derived neurotrophic factor. Hum Mol Genet (2007) 16(22):2659–68. doi: 10.1093/hmg/ddm189
124. Mirowska-Guzel D, Mach A, Gromadzka G, Czlonkowski A, Czlonkowska A. BDNF A196G and C270T gene polymorphisms and susceptibility to multiple sclerosis in the polish population. Gender Diff J Neuroimmunol (2008) 193(1):170–2. doi: 10.1016/j.jneuroim.2007.10.013
125. Huang R, Huang J, Cathcart H, Smith S, Poduslo SE. Genetic variants in brain-derived neurotrophic factor associated with Alzheimer’s disease. J Med Genet (2007) 44(2):e66. doi: 10.1136/jmg.2006.044883
126. Chen Z-Y, Jing D, Bath KG, Ieraci A, Khan T, Siao C-J, et al. Genetic Variant BDNF (Val66Met) Polymorphism Alters Anxiety-Related Behavior. Science (2006) 314(5796):140–3. doi: 10.1126/science.1129663
127. Egan MF, Kojima M, Callicott JH, Goldberg TE, Kolachana BS, Bertolino A, et al. The BDNF val66met Polymorphism Affects Activity-Dependent Secretion of BDNF and Human Memory and Hippocampal Function. Cell (2003) 112(2):257–69. doi: 10.1016/S0092-8674(03)00035-7
128. Reick C, Ellrichmann G, Tsai T, Lee D-H, Wiese S, Gold R, et al. Expression of brain-derived neurotrophic factor in astrocytes - Beneficial effects of glatiramer acetate in the R6/2 and YAC128 mouse models of Huntington’s disease. Exp Neurol (2016) 285(Pt A):12–23. doi: 10.1016/j.expneurol.2016.08.012
129. Colombo E, Cordiglieri C, Melli G, Newcombe J, Krumbholz M, Parada LF, et al. Stimulation of the neurotrophin receptor TrkB on astrocytes drives nitric oxide production and neurodegeneration. J Exp Med (2012) 209(3):521–35. doi: 10.1084/jem.20110698
130. Schwartz JP, Nishiyama N. Neurotrophic factor gene expression in astrocytes during development and following injury. Brain Res Bull (1994) 35(5):403–7. doi: 10.1016/0361-9230(94)90151-1
131. Micera A, Vigneti E, Aloe L. Changes of NGF presence in nonneuronal cells in response to experimental allergic encephalomyelitis in Lewis rats. Exp Neurol (1998) 154(1):41–6. doi: 10.1006/exnr.1998.6864
132. Goss JR, O’Malley ME, Zou L, Styren SD, Kochanek PM, DeKosky ST. Astrocytes are the major source of nerve growth factor upregulation following traumatic brain injury in the rat. Exp Neurol (1998) 149(2):301–9. doi: 10.1006/exnr.1997.6712
133. Iulita MF, Cuello AC. Nerve growth factor metabolic dysfunction in Alzheimer’s disease and Down syndrome. Trends Pharmacol Sci (2014) 35(7):338–48. doi: 10.1016/j.tips.2014.04.010
134. Beattie MS, Harrington AW, Lee R, Kim JY, Boyce SL, Longo FM, et al. ProNGF Induces p75-Mediated Death of Oligodendrocytes following Spinal Cord Injury. Neuron (2002) 36(3):375–86. doi: 10.1016/S0896-6273(02)01005-X
135. Marchetti L, Bonsignore F, Gobbo F, Amodeo R, Calvello M, Jacob A, et al. Fast-diffusing p75NTR monomers support apoptosis and growth cone collapse by neurotrophin ligands. Proc Natl Acad Sci U S A (2019) 116(43):21563–72. doi: 10.1073/pnas.1902790116
136. Tiveron C, Fasulo L, Capsoni S, Malerba F, Marinelli S, Paoletti F, et al. ProNGF\NGF imbalance triggers learning and memory deficits, neurodegeneration and spontaneous epileptic-like discharges in transgenic mice. Cell Death Differ (2013) 20(8):1017–30. doi: 10.1038/cdd.2013.22
137. Fahnestock M, Michalski B, Xu B, Coughlin MD. The precursor pro-nerve growth factor is the predominant form of nerve growth factor in brain and is increased in Alzheimer’s disease. Mol Cell Neurosci (2001) 18(2):210–20. doi: 10.1006/mcne.2001.1016
138. Fahnestock M, Shekari A. ProNGF and Neurodegeneration in Alzheimer’s Disease. Front Neurosci (2019) 13:129. doi: 10.3389/fnins.2019.00129
139. Friedman WJ, Thakur S, Seidman L, Rabson AB. Regulation of Nerve Growth Factor mRNA by Interleukin-1 in Rat Hippocampal Astrocytes Is Mediated by NFκB. J Biol Chem (1996) 271(49):31115–20. doi: 10.1074/jbc.271.49.31115
140. Cirillo G, Cavaliere C, Bianco MR, De Simone A, Colangelo AM, Sellitti S, et al. Intrathecal NGF administration reduces reactive astrocytosis and changes neurotrophin receptors expression pattern in a rat model of neuropathic pain. Cell Mol Neurobiol (2010) 30(1):51–62. doi: 10.1007/s10571-009-9430-2
141. Airaksinen MS, Saarma M. The GDNF family: Signalling, biological functions and therapeutic value. Nat Rev Neurosci (2002) 3(5):383–94. doi: 10.1038/nrn812
142. Baloh RH, Enomoto H, Johnson EM, Milbrandt J. The GDNF family ligands and receptors — implications for neural development. Curr Opin Neurobiol (2000) 10(1):103–10. doi: 10.1016/S0959-4388(99)00048-3
143. Peterziel H, Unsicker K, Krieglstein K. TGFβ induces GDNF responsiveness in neurons by recruitment of GFRα1 to the plasma membrane. J Cell Biol (2002) 159(1):157–67. doi: 10.1083/jcb.200203115
144. Paratcha G, Ledda F, Ibáñez CF. The neural cell adhesion molecule NCAM is an alternative signaling receptor for GDNF family ligands. Cell (2003) 113(7):867–79. doi: 10.1016/S0092-8674(03)00435-5
145. Erickson JT, Brosenitsch TA, Katz DM. Brain-derived neurotrophic factor and glial cell line-derived neurotrophic factor are required simultaneously for survival of dopaminergic primary sensory neurons in vivo. J Neurosci Off J Soc Neurosci (2001) 21(2):581–9. doi: 10.1523/JNEUROSCI.21-02-00581.2001
146. Tsui-Pierchala BA, Milbrandt J, Johnson EM. NGF utilizes c-Ret via a novel GFL-independent, inter-RTK signaling mechanism to maintain the trophic status of mature sympathetic neurons. Neuron (2002) 33(2):261–73. doi: 10.1016/S0896-6273(01)00585-2
147. Nielsen J, Gotfryd K, Li S, Kulahin N, Soroka V, Rasmussen KK, et al. Role of glial cell line-derived neurotrophic factor (GDNF)-neural cell adhesion molecule (NCAM) interactions in induction of neurite outgrowth and identification of a binding site for NCAM in the heel region of GDNF. J Neurosci Off J Soc Neurosci (2009) 29(36):11360–76. doi: 10.1523/JNEUROSCI.3239-09.2009
148. Klein SM, Behrstock S, McHugh J, Hoffmann K, Wallace K, Suzuki M, et al. GDNF Delivery Using Human Neural Progenitor Cells in a Rat Model of ALS. Hum Gene Ther (2005) 16(4):509–21. doi: 10.1089/hum.2005.16.509
149. Thomsen GM, Avalos P, Ma AA, Alkaslasi M, Cho N, Wyss L, et al. Transplantation of Neural Progenitor Cells Expressing Glial Cell Line-Derived Neurotrophic Factor into the Motor Cortex as a Strategy to Treat Amyotrophic Lateral Sclerosis. Stem Cells (2018) 36(7):1122–31. doi: 10.1002/stem.2825
150. Oppenheim RW, Prevette D, Yin QW, Collins F, MacDonald J. Control of embryonic motoneuron survival in vivo by ciliary neurotrophic factor. Science (1991) 251(5001):1616–8. doi: 10.1126/science.2011743
151. Hughes SM, Lillien LE, Raff MC, Rohrer H, Sendtner M. Ciliary neurotrophic factor induces type-2 astrocyte differentiation in culture. Nature (1988) 335(6185):70–3. doi: 10.1038/335070a0
152. Rowitch DH. Glial specification in the vertebrate neural tube. Nat Rev Neurosci (2004) 5(5):409–19. doi: 10.1038/nrn1389
153. Stankoff B, Aigrot M-S, Noël F, Wattilliaux A, Zalc B, Lubetzki C. Ciliary Neurotrophic Factor (CNTF) Enhances Myelin Formation: A Novel Role for CNTF and CNTF-Related Molecules. J Neurosci (2002) 22(21):9221–7. doi: 10.1523/JNEUROSCI.22-21-09221.2002
154. Martin A, Hofmann H-D, Kirsch M. Glial reactivity in ciliary neurotrophic factor-deficient mice after optic nerve lesion. J Neurosci Off J Soc Neurosci (2003) 23(13):5416–24. doi: 10.1523/JNEUROSCI.23-13-05416.2003
155. Stahl N, Yancopoulos GD. The tripartite CNTF receptor complex: activation and signaling involves components shared with other cytokines. J Neurobiol (1994) 25(11):1454–66. doi: 10.1002/neu.480251111
156. Bonni A, Frank DA, Schindler C, Greenberg ME. Characterization of a pathway for ciliary neurotrophic factor signaling to the nucleus. Science (1993) 262(5139):1575–9. doi: 10.1126/science.7504325
157. Dallner C, Woods AG, Deller T, Kirsch M, Hofmann H-D. CNTF and CNTF receptor alpha are constitutively expressed by astrocytes in the mouse brain. Glia (2002) 37(4):374–8. doi: 10.1002/glia.10048
158. Dutta R, McDonough J, Chang A, Swamy L, Siu A, Kidd GJ, et al. Activation of the ciliary neurotrophic factor (CNTF) signalling pathway in cortical neurons of multiple sclerosis patients. Brain J Neurol (2007) 130(Pt 10):2566–76. doi: 10.1093/brain/awm206
159. Gudi V, Škuljec J, Yildiz Ö, Frichert K, Skripuletz T, Moharregh-Khiabani D, et al. Spatial and temporal profiles of growth factor expression during CNS demyelination reveal the dynamics of repair priming. PloS One (2011) 6(7):e22623. doi: 10.1371/journal.pone.0022623
160. Kuhlmann T, Remington L, Cognet I, Bourbonniere L, Zehntner S, Guilhot F, et al. Continued Administration of Ciliary Neurotrophic Factor Protects Mice from Inflammatory Pathology in Experimental Autoimmune Encephalomyelitis. Am J Pathol (2006) 169(2):584–98. doi: 10.2353/ajpath.2006.051086
161. Petrova P, Raibekas A, Pevsner J, Vigo N, Anafi M, Moore MK, et al. MANF: a new mesencephalic, astrocyte-derived neurotrophic factor with selectivity for dopaminergic neurons. J Mol Neurosci MN (2003) 20(2):173–88. doi: 10.1385/JMN:20:2:173
162. Voutilainen MH, Bäck S, Pörsti E, Toppinen L, Lindgren L, Lindholm P, et al. Mesencephalic Astrocyte-Derived Neurotrophic Factor Is Neurorestorative in Rat Model of Parkinson’s Disease. J Neurosci (2009) 29(30):9651–9. doi: 10.1523/JNEUROSCI.0833-09.2009
163. Glembotski CC, Thuerauf DJ, Huang C, Vekich JA, Gottlieb RA, Doroudgar S. Mesencephalic astrocyte-derived neurotrophic factor protects the heart from ischemic damage and is selectively secreted upon sarco/endoplasmic reticulum calcium depletion. J Biol Chem (2012) 287(31):25893–904. doi: 10.1074/jbc.M112.356345
164. Lindahl M, Danilova T, Palm E, Lindholm P, Võikar V, Hakonen E, et al. MANF is indispensable for the proliferation and survival of pancreatic β cells. Cell Rep (2014) 7(2):366–75. doi: 10.1016/j.celrep.2014.03.023
165. Chen L, Feng L, Wang X, Du J, Chen Y, Yang W, et al. Mesencephalic astrocyte-derived neurotrophic factor is involved in inflammation by negatively regulating the NF-κB pathway. Sci Rep (2015) 5:8133. doi: 10.1038/srep08133
166. Liu J, Zhou C, Tao X, Feng L, Wang X, Chen L, et al. ER stress-inducible protein MANF selectively expresses in human spleen. Hum Immunol (2015) 76(11):823–30. doi: 10.1016/j.humimm.2015.09.043
167. Petrova PS, Raibekas A, Pevsner J, Vigo N, Anafi M, Moore MK, et al. MANF. J Mol Neurosci (2003) 20(2):173–87. doi: 10.1385/JMN:20:2:173
168. Lindholm P, Voutilainen MH, Laurén J, Peränen J, Leppänen V-M, Andressoo J-O, et al. Novel neurotrophic factor CDNF protects and rescues midbrain dopamine neurons in vivo. Nature (2007) 448(7149):73–7. doi: 10.1038/nature05957
169. Neves J, Zhu J, Sousa-Victor P, Konjikusic M, Riley R, Chew S, et al. Immune modulation by MANF promotes tissue repair and regenerative success in the retina. Science (2016) 353(6294):aaf3646. doi: 10.1126/science.aaf3646
170. Yan Y, Rato C, Rohland L, Preissler S, Ron D. MANF antagonizes nucleotide exchange by the endoplasmic reticulum chaperone BiP. Nat Commun (2019) 10(1):1–15. doi: 10.1038/s41467-019-08450-4
171. Arrieta A, Blackwood EA, Stauffer WT, Domingo MS, Bilal AS, Thuerauf DJ, et al. Mesencephalic astrocyte–derived neurotrophic factor is an ER-resident chaperone that protects against reductive stress in the heart. J Biol Chem (2020) 295(22):7566–83. doi: 10.1074/jbc.RA120.013345
172. Lee A-H, Iwakoshi NN, Glimcher LH. XBP-1 regulates a subset of endoplasmic reticulum resident chaperone genes in the unfolded protein response. Mol Cell Biol (2003) 23(21):7448–59. doi: 10.1128/MCB.23.21.7448-7459.2003
173. Mizobuchi N, Hoseki J, Kubota H, Toyokuni S, Nozaki J, Naitoh M, et al. ARMET is a Soluble ER Protein Induced by the Unfolded Protein Response via ERSE-II Element. Cell Struct Funct (2007) 32(1):41–50. doi: 10.1247/csf.07001
174. Apostolou A, Shen Y, Liang Y, Luo J, Fang S. Armet, a UPR-upregulated protein, inhibits cell proliferation and ER stress-induced cell death. Exp Cell Res (2008) 314(13):2454–67. doi: 10.1016/j.yexcr.2008.05.001
175. Shim AH-R, Liu H, Focia PJ, Chen X, Lin PC, He X. Structures of a platelet-derived growth factor/propeptide complex and a platelet-derived growth factor/receptor complex. Proc Natl Acad Sci (2010) 107(25):11307–12. doi: 10.1073/pnas.1000806107
176. Coughlin SR, Moskowitz MA, Zetter BR, Antoniades HN, Levine L. Platelet-dependent stimulation of prostacyclin synthesis by platelet-derived growth factor. Nature (1980) 288(5791):600–2. doi: 10.1038/288600a0
177. Calver AR, Hall AC, Yu WP, Walsh FS, Heath JK, Betsholtz C, et al. Oligodendrocyte population dynamics and the role of PDGF in vivo. Neuron (1998) 20(5):869–82. doi: 10.1016/S0896-6273(00)80469-9
178. Đang TC, Ishii Y, Nguyen VD, Yamamoto S, Hamashima T, Okuno N, et al. Powerful Homeostatic Control of Oligodendroglial Lineage by PDGFRα in Adult Brain. Cell Rep (2019) 27(4):1073–1089.e5. doi: 10.1016/j.celrep.2019.03.084
179. Tao C, Zhang X. Directed migration of retinal astrocytes by PDGF signaling. Acta Ophthalmol (Copenh) (2017) 95(S259). doi: 10.1111/j.1755-3768.2017.02786
180. Fruttiger M, Calver AR, Krüger WH, Mudhar HS, Michalovich D, Takakura N, et al. PDGF Mediates a Neuron–Astrocyte Interaction in the Developing Retina. Neuron (1996) 17(6):1117–31. doi: 10.1016/S0896-6273(00)80244-5
181. Pringle N, Collarini EJ, Mosley MJ, Heldin CH, Westermark B, Richardson WD. PDGF A chain homodimers drive proliferation of bipotential (O-2A) glial progenitor cells in the developing rat optic nerve. EMBO J (1989) 8(4):1049–56. doi: 10.1002/j.1460-2075.1989.tb03472.x
182. Silberstein FC, De Simone R, Levi G, Aloisi F. Cytokine-regulated expression of platelet-derived growth factor gene and protein in cultured human astrocytes. J Neurochem (1996) 66(4):1409–17. doi: 10.1046/j.1471-4159.1996.66041409.x
183. Kernt M, Liegl RG, Rueping J, Neubauer AS, Haritoglou C, Lackerbauer CA, et al. Sorafenib protects human optic nerve head astrocytes from light-induced overexpression of vascular endothelial growth factor, platelet-derived growth factor, and placenta growth factor. Growth Factors Chur Switz (2010) 28(3):211–20. doi: 10.3109/08977191003604505
184. Richardson WD, Pringle N, Mosley MJ, Westermark B, Dubois-Dalcq M. A role for platelet-derived growth factor in normal gliogenesis in the central nervous system. Cell (1988) 53(2):309–19. doi: 10.1016/0092-8674(88)90392-3
185. Moore CS, Abdullah SL, Brown A, Arulpragasam A, Crocker SJ. How factors secreted from astrocytes impact myelin repair. J Neurosci Res (2011) 89(1):13–21. doi: 10.1002/jnr.22482
186. Durand B, Raff M. A cell-intrinsic timer that operates during oligodendrocyte development. BioEssays News Rev Mol Cell Dev Biol (2000) 22(1):64–71. doi: 10.1002/(SICI)1521-1878(200001)22:1<64::AID-BIES11>3.0.CO;2-Q
187. Giacobini MM, Almström S, Funa K, Olson L. Differential effects of platelet-derived growth factor isoforms on dopamine neurons in vivo: -BB supports cell survival, -AA enhances fiber formation. Neuroscience (1993) 57(4):923–9. doi: 10.1016/0306-4522(93)90038-H
188. Erlandsson A, Enarsson M, Forsberg-Nilsson K. Immature Neurons From CNS Stem Cells Proliferate in Response to Platelet-Derived Growth Factor. J Neurosci (2001) 21(10):3483–91. doi: 10.1523/JNEUROSCI.21-10-03483.2001
189. Yeh H-J, Ruit KG, Wang Y-X, Parks WC, Snider WD, Deuel TF. PDGF A-chain gene is expressed by mammalian neurons during development and in maturity. Cell (1991) 64(1):209–16. doi: 10.1016/0092-8674(91)90222-K
190. Sasahara M, Fries JWU, Raines EW, Gown AM, Westrum LE, Frosch MP, et al. PDGF B-chain in neurons of the central nervous system, posterior pituitary, and in a transgenic model. Cell (1991) 64(1):217–27. doi: 10.1016/0092-8674(91)90223-L
191. Funa K, Sasahara M. The Roles of PDGF in Development and During Neurogenesis in the Normal and Diseased Nervous System. J Neuroimmune Pharmacol (2014) 9(2):168–81. doi: 10.1007/s11481-013-9479-z
192. Rash BG, Tomasi S, Lim HD, Suh CY, Vaccarino FM. Cortical Gyrification Induced by Fibroblast Growth Factor 2 in the Mouse Brain. J Neurosci (2013) 33(26):10802–14. doi: 10.1523/JNEUROSCI.3621-12.2013
193. Noda M, Takii K, Parajuli B, Kawanokuchi J, Sonobe Y, Takeuchi H, et al. FGF-2 released from degenerating neurons exerts microglial-induced neuroprotection via FGFR3-ERK signaling pathway. J Neuroinflam (2014) 11(1):1–11. doi: 10.1186/1742-2094-11-76
194. Diez del Corral R, Morales AV. The Multiple Roles of FGF Signaling in the Developing Spinal Cord. Front Cell Dev Biol (2017) 5:58. doi: 10.3389/fcell.2017.00058
195. Goetz R, Mohammadi M. Exploring mechanisms of FGF signalling through the lens of structural biology. Nat Rev Mol Cell Biol (2013) 14(3):166–80. doi: 10.1038/nrm3528
196. Duong TAD, Hoshiba Y, Saito K, Kawasaki K, Ichikawa Y, Matsumoto N, et al. FGF Signaling Directs the Cell Fate Switch from Neurons to Astrocytes in the Developing Mouse Cerebral Cortex. J Neurosci (2019) 39(31):6081–94. doi: 10.1523/JNEUROSCI.2195-18.2019
197. Stork T, Sheehan A, Tasdemir-Yilmaz OE, Freeman MR. Neuron-glia interactions through the Heartless FGF receptor signaling pathway mediate morphogenesis of Drosophila astrocytes. Neuron (2014) 83(2):388–403. doi: 10.1016/j.neuron.2014.06.026
198. Savchenko E, Teku GN, Boza-Serrano A, Russ K, Berns M, Deierborg T, et al. FGF family members differentially regulate maturation and proliferation of stem cell-derived astrocytes. Sci Rep (2019) 9(1):9610. doi: 10.1038/s41598-019-46110-1
199. Kang W, Balordi F, Su N, Chen L, Fishell G, Hébert JM. Astrocyte activation is suppressed in both normal and injured brain by FGF signaling. Proc Natl Acad Sci (2014) 111(29):E2987–95. doi: 10.1073/pnas.1320401111
200. Reuss B, von Bohlen und Halbach O. Fibroblast growth factors and their receptors in the central nervous system. Cell Tissue Res (2003) 313(2):139–57. doi: 10.1007/s00441-003-0756-7
201. Garel S, Huffman KJ, Rubenstein JLR. Molecular regionalization of the neocortex is disrupted in Fgf8 hypomorphic mutants. Development (2003) 130(9):1903–14. doi: 10.1242/dev.00416
202. Fukuchi-Shimogori T, Grove EA. Neocortex Patterning by the Secreted Signaling Molecule FGF8. Science (2001) 294(5544):1071–4. doi: 10.1126/science.1064252
203. Rash BG, Lim HD, Breunig JJ, Vaccarino FM. FGF Signaling Expands Embryonic Cortical Surface Area by Regulating Notch-Dependent Neurogenesis. J Neurosci (2011) 31(43):15604–17. doi: 10.1523/JNEUROSCI.4439-11.2011
204. Mohan H, Friese A, Albrecht S, Krumbholz M, Elliott CL, Arthur A, et al. Transcript profiling of different types of multiple sclerosis lesions yields FGF1 as a promoter of remyelination. Acta Neuropathol Commun (2014) 2(1):168. doi: 10.1186/s40478-014-0168-9
205. Teh DBL, Prasad A, Jiang W, Ariffin MZ, Khanna S, Belorkar A, et al. Transcriptome Analysis Reveals Neuroprotective aspects of Human Reactive Astrocytes induced by Interleukin 1β. Sci Rep (2017) 7(1):1–12. doi: 10.1038/s41598-017-13174-w
206. Fortin D, Rom E, Sun H, Yayon A, Bansal R. Distinct Fibroblast Growth Factor (FGF)/FGF Receptor Signaling Pairs Initiate Diverse Cellular Responses in the Oligodendrocyte Lineage. J Neurosci (2005) 25(32):7470–9. doi: 10.1523/JNEUROSCI.2120-05.2005
207. Okada T, Enkhjargal B, Travis ZD, Ocak U, Tang J, Suzuki H, et al. FGF-2 Attenuates Neuronal Apoptosis via FGFR3/PI3k/Akt Signaling Pathway After Subarachnoid Hemorrhage. Mol Neurobiol (2019) 56(12):8203–19. doi: 10.1007/s12035-019-01668-9
208. Ruffini F, Furlan R, Poliani PL, Brambilla E, Marconi PC, Bergami A, et al. Fibroblast growth factor-II gene therapy reverts the clinical course and the pathological signs of chronic experimental autoimmune encephalomyelitis in C57BL/6 mice. Gene Ther (2001) 8(16):1207–13. doi: 10.1038/sj.gt.3301523
209. Thümmler K, Rom E, Zeis T, Lindner M, Brunner S, Cole JJ, et al. Polarizing receptor activation dissociates fibroblast growth factor 2 mediated inhibition of myelination from its neuroprotective potential. Acta Neuropathol Commun (2019) 7(1):212. doi: 10.1186/s40478-019-0864-6
210. Tobin JE, Xie M, Le TQ, Song S-K, Armstrong RC. Reduced Axonopathy and Enhanced Remyelination After Chronic Demyelination in Fibroblast Growth Factor 2 (Fgf2)-Null Mice: Differential Detection With Diffusion Tensor Imaging. J Neuropathol Exp Neurol (2011) 70(2):157–65. doi: 10.1097/NEN.0b013e31820937e4
211. Fressinaud C, Vallat JM, Labourdette G. Basic fibroblast growth factor down-regulates myelin basic protein gene expression and alters myelin compaction of mature oligodendrocytes in vitro. J Neurosci Res (1995) 40(3):285–93. doi: 10.1002/jnr.490400302
212. Lindner M, Thümmler K, Arthur A, Brunner S, Elliott C, McElroy D, et al. Fibroblast growth factor signalling in multiple sclerosis: inhibition of myelination and induction of pro-inflammatory environment by FGF9. Brain (2015) 138(7):1875–93. doi: 10.1093/brain/awv102
213. Sarchielli P, Di Filippo M, Ercolani MV, Chiasserini D, Mattioni A, Bonucci M, et al. Fibroblast growth factor-2 levels are elevated in the cerebrospinal fluid of multiple sclerosis patients. Neurosci Lett (2008) 435(3):223–8. doi: 10.1016/j.neulet.2008.02.040
214. Higashiyama S, Abraham JA, Miller J, Fiddes JC, Klagsbrun M. A heparin-binding growth factor secreted by macrophage-like cells that is related to EGF. Science (1991) 251(4996):936–9. doi: 10.1126/science.1840698
215. Nishi E, Klagsbrun M. Heparin-binding epidermal growth factor-like growth factor (HB-EGF) is a mediator of multiple physiological and pathological pathways. Growth Factors Chur Switz (2004) 22(4):253–60. doi: 10.1080/08977190400008448
216. Yang Z, Mosser DM, Zhang X. Activation of the MAPK, ERK, following Leishmania amazonensis Infection of Macrophages. J Immunol (2007) 178(2):1077–85. doi: 10.4049/jimmunol.178.2.1077
217. Edwards JP, Zhang X, Mosser DM. The Expression of Heparin-Binding Epidermal Growth Factor-Like Growth Factor by Regulatory Macrophages. J Immunol Baltim Md (1950) 182(4):1929–39. doi: 10.4049/jimmunol.0802703
218. Shen Y, Ruan L, Lian C, Li R, Tu Z, Liu H. Discovery of HB-EGF binding peptides and their functional characterization in ovarian cancer cell lines. Cell Death Discovery (2019) 5(1):1–11. doi: 10.1038/s41420-019-0163-9
219. Miyata K, Yotsumoto F, Nam SO, Kuroki M, Miyamoto S. Regulatory Mechanisms of the HB-EGF Autocrine Loop in Inflammation, Homeostasis, Development and Cancer. Anticancer Res (2012) 32(6):2347–52.
220. Mehta VB, Besner GE. Heparin-Binding Epidermal Growth Factor-Like Growth Factor Inhibits Cytokine-Induced NF-κB Activation and Nitric Oxide Production via Activation of the Phosphatidylinositol 3-Kinase Pathway. J Immunol (2005) 175(3):1911–8. doi: 10.4049/jimmunol.175.3.1911
221. Farahnak S, Simon L, McGovern TK, Chen M, Khazaei N, Martin JG. HB-EGF Synthesized by CD4 T Cells Modulates Allergic Airway Eosinophilia by Regulating IL-5 Synthesis. J Immunol (2019) 203:39–47. doi: 10.4049/jimmunol.1801686
222. Mehta VB, Besner GE. Inhibition of NF-κB Activation and Its Target Genes by Heparin-Binding Epidermal Growth Factor-Like Growth Factor. J Immunol (2003) 171(11):6014–22. doi: 10.4049/jimmunol.171.11.6014
223. Jin K, Mao XO, Sun Y, Xie L, Jin L, Nishi E, et al. Heparin-binding epidermal growth factor-like growth factor: hypoxia-inducible expression in vitro and stimulation of neurogenesis in vitro and in vivo. J Neurosci Off J Soc Neurosci (2002) 22(13):5365–73. doi: 10.1523/JNEUROSCI.22-13-05365.2002
224. Farkas LM, Krieglstein K. Heparin-binding epidermal growth factor-like growth factor (HB-EGF) regulates survival of midbrain dopaminergic neurons. J Neural Transm Vienna Austria 1996 (2002) 109(3):267–77. doi: 10.1007/s007020200022
225. Wang L-M, Kuo A, Alimandi M, Veri MC, Lee C-C, Kapoor V, et al. ErbB2 expression increases the spectrum and potency of ligand-mediated signal transduction through ErbB4. Proc Natl Acad Sci (1998) 95(12):6809–14. doi: 10.1073/pnas.95.12.6809
226. Nishi E, Prat A, Hospital V, Elenius K, Klagsbrun M. N-arginine dibasic convertase is a specific receptor for heparin-binding EGF-like growth factor that mediates cell migration. EMBO J (2001) 20(13):3342–50. doi: 10.1093/emboj/20.13.3342
227. Iwamoto R, Yamazaki S, Asakura M, Takashima S, Hasuwa H, Miyado K, et al. Heparin-binding EGF-like growth factor and ErbB signaling is essential for heart function. Proc Natl Acad Sci (2003) 100(6):3221–6. doi: 10.1073/pnas.0537588100
228. Clayton PE, Banerjee I, Murray PG, Renehan AG. Growth hormone, the insulin-like growth factor axis, insulin and cancer risk. Nat Rev Endocrinol (2011) 7(1):11–24. doi: 10.1038/nrendo.2010.171
229. Fernandez AM, Torres-Alemán I. The many faces of insulin-like peptide signalling in the brain. Nat Rev Neurosci (2012) 13(4):225–39. doi: 10.1038/nrn3209
230. Song Y, Pimentel C, Walters K, Boller L, Ghiasvand S, Liu J, et al. Neuroprotective levels of IGF-1 exacerbate epileptogenesis after brain injury. Sci Rep (2016) 6(1):32095. doi: 10.1038/srep32095
231. Cohen E, Dillin A. The insulin paradox: aging, proteotoxicity and neurodegeneration. Nat Rev Neurosci (2008) 9(10):759–67. doi: 10.1038/nrn2474
232. Sehat B, Tofigh A, Lin Y, Trocmé E, Liljedahl U, Lagergren J, et al. SUMOylation mediates the nuclear translocation and signaling of the IGF-1 receptor. Sci Signal (2010) 3(108):ra10. doi: 10.1126/scisignal.2000628
233. Beilharz EJ, Russo VC, Butler G, Baker NL, Connor B, Sirimanne ES, et al. Co-ordinated and cellular specific induction of the components of the IGF/IGFBP axis in the rat brain following hypoxic-ischemic injury. Brain Res Mol Brain Res (1998) 59(2):119–34. doi: 10.1016/S0169-328X(98)00122-3
234. Walter HJ, Berry M, Hill DJ, Cwyfan-Hughes S, Holly JM, Logan A. Distinct sites of insulin-like growth factor (IGF)-II expression and localization in lesioned rat brain: possible roles of IGF binding proteins (IGFBPs) in the mediation of IGF-II activity. Endocrinology (1999) 140(1):520–32. doi: 10.1210/endo.140.1.6463
235. Li MO, Flavell RA. TGF-beta: a master of all T cell trades. Cell (2008) 134(3):392–404. doi: 10.1016/j.cell.2008.07.025
236. Li MO, Wan YY, Sanjabi S, Robertson A-KL, Flavell RA. TRANSFORMING GROWTH FACTOR-β REGULATION OF IMMUNE RESPONSES. Annu Rev Immunol (2006) 24(1):99–146. doi: 10.1146/annurev.immunol.24.021605.090737
237. Li MO, Flavell RA. Contextual Regulation of Inflammation: A Duet by Transforming Growth Factor-β and Interleukin-10. Immunity (2008) 28(4):468–76. doi: 10.1016/j.immuni.2008.03.003
238. Huse M, Muir TW, Xu L, Chen Y-G, Kuriyan J, Massagué J. The TGFβ Receptor Activation Process: An Inhibitor- to Substrate-Binding Switch. Mol Cell (2001) 8(3):671–82. doi: 10.1016/S1097-2765(01)00332-X
239. Shull MM, Ormsby I, Kier AB, Pawlowski S, Diebold RJ, Yin M, et al. Targeted disruption of the mouse transforming growth factor-beta 1 gene results in multifocal inflammatory disease. Nature (1992) 359(6397):693–9. doi: 10.1038/359693a0
240. Gorelik L, Flavell RA. Transforming growth factor-β in T-cell biology. Nat Rev Immunol (2002) 2(1):46–53. doi: 10.1038/nri704
241. Meyers EA, Kessler JA. TGF-β Family Signaling in Neural and Neuronal Differentiation, Development, and Function. Cold Spring Harb Perspect Biol (2017) 9(8):a022244. doi: 10.1101/cshperspect.a022244
242. Dünker N, Krieglstein K. Reduced programmed cell death in the retina and defects in lens and cornea of Tgfβ2–/–Tgfβ3–/–double-deficient mice. Cell Tissue Res (2003) 313(1):1–10. doi: 10.1007/s00441-003-0761-x
243. Krieglstein K, Unsicker K. Transforming growth factor-β promotes survival of midbrain dopaminergic neurons and protects them against N-methyl-4-phenylpyridinium ion toxicity. Neuroscience (1994) 63(4):1189–96. doi: 10.1016/0306-4522(94)90583-5
244. Krieglstein K, Henheik P, Farkas L, Jaszai J, Galter D, Krohn K, et al. Glial Cell Line-Derived Neurotrophic Factor Requires Transforming Growth Factor-β for Exerting Its Full Neurotrophic Potential on Peripheral and CNS Neurons. J Neurosci (1998) 18(23):9822–34. doi: 10.1523/JNEUROSCI.18-23-09822.1998
245. Krieglstein K, Richter S, Farkas L, Schuster N, Dünker N, Oppenheim RW, et al. Reduction of endogenous transforming growth factors β prevents ontogenetic neuron death. Nat Neurosci (2000) 3(11):1085–90. doi: 10.1038/80598
246. Kim SY, Senatorov VV, Morrissey CS, Lippmann K, Vazquez O, Milikovsky DZ, et al. TGFβ signaling is associated with changes in inflammatory gene expression and perineuronal net degradation around inhibitory neurons following various neurological insults. Sci Rep (2017) 7(1):1–14. doi: 10.1038/s41598-017-07394-3
247. Brionne TC, Tesseur I, Masliah E, Wyss-Coray T. Loss of TGF-β1 Leads to Increased Neuronal Cell Death and Microgliosis in Mouse Brain. Neuron (2003) 40(6):1133–45. doi: 10.1016/S0896-6273(03)00766-9
248. Zhu Y, Yang G-Y, Ahlemeyer B, Pang L, Che X-M, Culmsee C, et al. Transforming Growth Factor-β1 Increases Bad Phosphorylation and Protects Neurons Against Damage. J Neurosci (2002) 22(10):3898–909. doi: 10.1523/JNEUROSCI.22-10-03898.2002
249. Xue X, Zhang W, Zhu J, Chen X, Zhou S, Xu Z, et al. Aquaporin-4 deficiency reduces TGF-β1 in mouse midbrains and exacerbates pathology in experimental Parkinson’s disease. J Cell Mol Med (2019) 23(4):2568–82. doi: 10.1111/jcmm.14147
250. Cekanaviciute E, Fathali N, Doyle KP, Williams AM, Han J, Buckwalter MS. Astrocytic transforming growth factor-beta signaling reduces subacute neuroinflammation after stroke in mice. Glia (2014) 62(8):1227–40. doi: 10.1002/glia.22675
251. Diniz LP, Tortelli V, Matias I, Morgado J, Araujo APB, Melo HM, et al. Astrocyte Transforming Growth Factor Beta 1 Protects Synapses against Aβ Oligomers in Alzheimer’s Disease Model. J Neurosci (2017) 37(28):6797–809. doi: 10.1523/JNEUROSCI.3351-16.2017
252. Ni H, Ding N-Z, Harper MJK, Yang Z-M. Expression of leukemia inhibitory factor receptor and gp130 in mouse uterus during early pregnancy. Mol Reprod Dev (2002) 63(2):143–50. doi: 10.1002/mrd.10168
253. Gearing DP, Gough NM, King JA, Hilton DJ, Nicola NA, Simpson RJ, et al. Molecular cloning and expression of cDNA encoding a murine myeloid leukaemia inhibitory factor (LIF). EMBO J (1987) 6(13):3995–4002. doi: 10.1002/j.1460-2075.1987.tb02742.x
254. Wang H, Wang J, Zhao Y, Zhang X, Liu J, Zhang C, et al. LIF is essential for ISC function and protects against radiation-induced gastrointestinal syndrome. Cell Death Dis (2020) 11(7):1–15. doi: 10.1038/s41419-020-02790-6
255. Yu H, Yue X, Zhao Y, Li X, Wu L, Zhang C, et al. LIF negatively regulates tumour-suppressor p53 through Stat3/ID1/MDM2 in colorectal cancers. Nat Commun (2014) 5(1):1–12. doi: 10.1038/ncomms6218
256. Pascual-García M, Bonfill-Teixidor E, Planas-Rigol E, Rubio-Perez C, Iurlaro R, Arias A, et al. LIF regulates CXCL9 in tumor-associated macrophages and prevents CD8 + T cell tumor-infiltration impairing anti-PD1 therapy. Nat Commun (2019) 10(1):1–11. doi: 10.1038/s41467-019-10369-9
257. Nguyen HN, Noss EH, Mizoguchi F, Huppertz C, Wei KS, Watts GFM, et al. Autocrine Loop Involving IL-6 Family Member LIF, LIF Receptor, and STAT4 Drives Sustained Fibroblast Production of Inflammatory Mediators. Immunity (2017) 46(2):220–32. doi: 10.1016/j.immuni.2017.01.004
258. Scott RL, Gurusinghe AD, Rudvosky AA, Kozlakivsky V, Murray SS, Satoh M, et al. Expression of leukemia inhibitory factor receptor mRNA in sensory dorsal root ganglion and spinal motor neurons of the neonatal rat. Neurosci Lett (2000) 295(1):49–53. doi: 10.1016/S0304-3940(00)01578-0
259. Bauer S, Kerr BJ, Patterson PH. The neuropoietic cytokine family in development, plasticity, disease and injury. Nat Rev Neurosci (2007) 8(3):221–32. doi: 10.1038/nrn2054
260. Moon C, Yoo J-Y, Matarazzo V, Sung YK, Kim EJ, Ronnett GV. Leukemia inhibitory factor inhibits neuronal terminal differentiation through STAT3 activation. Proc Natl Acad Sci (2002) 99(13):9015–20. doi: 10.1073/pnas.132131699
261. Shimazaki T, Shingo T, Weiss S. The ciliary neurotrophic factor/leukemia inhibitory factor/gp130 receptor complex operates in the maintenance of mammalian forebrain neural stem cells. J Neurosci Off J Soc Neurosci (2001) 21(19):7642–53. doi: 10.1523/JNEUROSCI.21-19-07642.2001
262. Bauer S, Patterson PH. Leukemia inhibitory factor promotes neural stem cell self-renewal in the adult brain. J Neurosci Off J Soc Neurosci (2006) 26(46):12089–99. doi: 10.1523/JNEUROSCI.3047-06.2006
263. Banner LR, Moayeri NN, Patterson PH. Leukemia inhibitory factor is expressed in astrocytes following cortical brain injury. Exp Neurol (1997) 147(1):1–9. doi: 10.1006/exnr.1997.6536
264. Suzuki S, Tanaka K, Nogawa S, Ito D, Dembo T, Kosakai A, et al. Immunohistochemical detection of leukemia inhibitory factor after focal cerebral ischemia in rats. J Cereb Blood Flow Metab Off J Int Soc Cereb Blood Flow Metab (2000) 20(4):661–8. doi: 10.1097/00004647-200004000-00003
265. Leibinger M, Müller A, Andreadaki A, Hauk TG, Kirsch M, Fischer D. Neuroprotective and Axon Growth-Promoting Effects following Inflammatory Stimulation on Mature Retinal Ganglion Cells in Mice Depend on Ciliary Neurotrophic Factor and Leukemia Inhibitory Factor. J Neurosci (2009) 29(45):14334–41. doi: 10.1523/JNEUROSCI.2770-09.2009
266. Emery B, Cate HS, Marriott M, Merson T, Binder MD, Snell C, et al. Suppressor of cytokine signaling 3 limits protection of leukemia inhibitory factor receptor signaling against central demyelination. Proc Natl Acad Sci U S A (2006) 103(20):7859–64. doi: 10.1073/pnas.0602574103
267. Gao W, Thompson L, Zhou Q, Putheti P, Fahmy TM, Strom TB, et al. Treg versus Th17 lymphocyte lineages are cross-regulated by LIF versus IL-6. Cell Cycle Georget Tex (2009) 8(9):1444–50. doi: 10.4161/cc.8.9.8348
268. Janssens K, Van den Haute C, Baekelandt V, Lucas S, van Horssen J, Somers V, et al. Leukemia inhibitory factor tips the immune balance towards regulatory T cells in multiple sclerosis. Brain Behav Immun (2015) 45:180–8. doi: 10.1016/j.bbi.2014.11.010
269. Metcalfe SM. LIF in the regulation of T-cell fate and as a potential therapeutic. Genes Immun (2011) 12(3):157–68. doi: 10.1038/gene.2011.9
270. Wiese S, Karus M, Faissner A. Astrocytes as a Source for Extracellular Matrix Molecules and Cytokines. Front Pharmacol (2012) 3. doi: 10.3389/fphar.2012.00120
271. Chao C-C, Gutiérrez-Vázquez C, Rothhammer V, Mayo L, Wheeler MA, Tjon EC, et al. Metabolic Control of Astrocyte Pathogenic Activity via cPLA2-MAVS. Cell (2019) 179(7):1483–98.e22. doi: 10.1016/j.cell.2019.11.016
272. Cooper ML, Pasini S, Lambert WS, D’Alessandro KB, Yao V, Risner ML, et al. Redistribution of metabolic resources through astrocyte networks mitigates neurodegenerative stress. Proc Natl Acad Sci (2020) 117(31):18810–21. doi: 10.1073/pnas.2009425117
273. Valenza M, Marullo M, Di Paolo E, Cesana E, Zuccato C, Biella G, et al. Disruption of astrocyte-neuron cholesterol cross talk affects neuronal function in Huntington’s disease. Cell Death Differ (2015) 22(4):690–702. doi: 10.1038/cdd.2014.162
274. Silver J, Miller JH. Regeneration beyond the glial scar. Nat Rev Neurosci (2004) 5(2):146–56. doi: 10.1038/nrn1326
275. Yong VW. Metalloproteinases: Mediators of Pathology and Regeneration in the CNS. Nat Rev Neurosci (2005) 6(12):931–44. doi: 10.1038/nrn1807
276. Lau LW, Cua R, Keough MB, Haylock-Jacobs S, Yong VW. Pathophysiology of the brain extracellular matrix: a new target for remyelination. Nat Rev Neurosci (2013) 14(10):722–9. doi: 10.1038/nrn3550
277. Yin K-J, Cirrito JR, Yan P, Hu X, Xiao Q, Pan X, et al. Matrix Metalloproteinases Expressed by Astrocytes Mediate Extracellular Amyloid-β Peptide Catabolism. J Neurosci (2006) 26(43):10939–48. doi: 10.1523/JNEUROSCI.2085-06.2006
278. Allen M, Ghosh S, Ahern GP, Villapol S, Maguire-Zeiss KA, Conant K. Protease induced plasticity: matrix metalloproteinase-1 promotes neurostructural changes through activation of protease activated receptor 1. Sci Rep (2016) 6(1):1–17. doi: 10.1038/srep35497
279. Banks WA. From blood–brain barrier to blood–brain interface: new opportunities for CNS drug delivery. Nat Rev Drug Discovery (2016) 15(4):275–92. doi: 10.1038/nrd.2015.21
280. Colombo E, Bassani C, De Angelis A, Ruffini F, Ottoboni L, Comi G, et al. Siponimod (BAF312) Activates Nrf2 While Hampering NFκB in Human Astrocytes, and Protects From Astrocyte-Induced Neurodegeneration. Front Immunol (2020) 11:635. doi: 10.3389/fimmu.2020.00635
281. Wu C, Leong SY, Moore CS, Cui QL, Gris P, Bernier L-P, et al. Dual effects of daily FTY720 on human astrocytes in vitro : relevance for neuroinflammation. J Neuroinflam (2013) 10(1):1–11. doi: 10.1186/1742-2094-10-41
282. Faissner S, Gold R. Oral Therapies for Multiple Sclerosis. Cold Spring Harb Perspect Med (2019) 9(1):a032011. doi: 10.1101/cshperspect.a032011
283. Vollmer TL, Sorensen PS, Selmaj K, Zipp F, Havrdova E, Cohen JA, et al. A randomized placebo-controlled phase III trial of oral laquinimod for multiple sclerosis. J Neurol (2014) 261(4):773–83. doi: 10.1007/s00415-014-7264-4
284. Comi G, Pulizzi A, Rovaris M, Abramsky O, Arbizu T, Boiko A, et al. Effect of laquinimod on MRI-monitored disease activity in patients with relapsing-remitting multiple sclerosis: a multicentre, randomised, double-blind, placebo-controlled phase IIb study. Lancet (2008) 371(9630):2085–92. doi: 10.1016/S0140-6736(08)60918-6
285. Steinman L. No quiet surrender: molecular guardians in multiple sclerosis brain. J Clin Invest (2015) 125(4):1371–8. doi: 10.1172/JCI74255
286. Safavi M, Abdollahi SN, Abdollahi M. Systematic Review of Drugs in Late-Stage Development for the Treatment of Multiple Sclerosis: A Focus on Oral Synthetic Drugs. Inflammation Allergy Drug Targets Discontin (2014) 13:351. doi: 10.2174/1871528114666150529102613
287. Hamby ME, Sofroniew MV. Reactive astrocytes as therapeutic targets for CNS disorders. Neurotherapeutics (2010) 7(4):494–506. doi: 10.1016/j.nurt.2010.07.003
288. Valori CF, Guidotti G, Brambilla L, Rossi D. Astrocytes: Emerging Therapeutic Targets in Neurological Disorders. Trends Mol Med (2019) 25(9):750–9. doi: 10.1016/j.molmed.2019.04.010
289. Anderson MA, Ao Y, Sofroniew MV. Heterogeneity of reactive astrocytes. Neurosci Lett (2014) 565:23–9. doi: 10.1016/j.neulet.2013.12.030
290. Khakh BS, Deneen B. The Emerging Nature of Astrocyte Diversity. Annu Rev Neurosci (2019) 42(1):187–207. doi: 10.1146/annurev-neuro-070918-050443
291. Wheeler MA, Clark IC, Tjon EC, Li Z, Zandee SEJ, Couturier CP, et al. MAFG-driven astrocytes promote CNS inflammation. Nature (2020) 578:593–9. doi: 10.1038/s41586-020-1999-0
292. Bayraktar OA, Bartels T, Holmqvist S, Kleshchevnikov V, Martirosyan A, Polioudakis D, et al. Astrocyte layers in the mammalian cerebral cortex revealed by a single-cell in situ transcriptomic map. Nat Neurosci (2020) 23(4):500–9. doi: 10.1038/s41593-020-0602-1
293. Batiuk MY, Martirosyan A, Wahis J, de Vin F, Marneffe C, Kusserow C, et al. Identification of region-specific astrocyte subtypes at single cell resolution. Nat Commun (2020) 11(1):1–15. doi: 10.1038/s41467-019-14198-8
294. Foust KD, Nurre E, Montgomery CL, Hernandez A, Chan CM, Kaspar BK. Intravascular AAV9 preferentially targets neonatal neurons and adult astrocytes. Nat Biotechnol (2009) 27(1):59–65. doi: 10.1038/nbt.1515
295. Samaranch L, Salegio EA, San Sebastian W, Kells AP, Foust KD, Bringas JR, et al. Adeno-Associated Virus Serotype 9 Transduction in the Central Nervous System of Nonhuman Primates. Hum Gene Ther (2011) 23(4):382–9. doi: 10.1089/hum.2011.200
296. von Jonquieres G, Mersmann N, Klugmann CB, Harasta AE, Lutz B, Teahan O, et al. Glial Promoter Selectivity following AAV-Delivery to the Immature Brain. PloS One (2013) 8(6):e65646. doi: 10.1371/journal.pone.0065646
297. Meng X, Yang F, Ouyang T, Liu B, Wu C, Jiang W. Specific gene expression in mouse cortical astrocytes is mediated by a 1740bp-GFAP promoter-driven combined adeno-associated virus2/5/7/8/9. Neurosci Lett (2015) 593:45–50. doi: 10.1016/j.neulet.2015.03.022
298. Kordower JH, Freeman TB, Snow BJ, Vingerhoets FJG, Mufson EJ, Sanberg PR, et al. Neuropathological Evidence of Graft Survival and Striatal Reinnervation after the Transplantation of Fetal Mesencephalic Tissue in a Patient with Parkinson’s Disease. N Engl J Med (1995) 332:1118–24. doi: 10.1056/NEJM199504273321702
299. Piccini P, Brooks DJ, Björklund A, Gunn RN, Grasby PM, Rimoldi O, et al. Dopamine release from nigral transplants visualized in vivo in a Parkinson’s patient. Nat Neurosci (1999) 2(12):1137–40. doi: 10.1038/16060
300. Piccini P, Lindvall O, Björklund A, Brundin P, Hagell P, Ceravolo R, et al. Delayed recovery of movement-related cortical function in Parkinson’s disease after striatal dopaminergic grafts. Ann Neurol (2000) 48(5):689–95. doi: 10.1002/1531-8249(200011)48:5<689::AID-ANA1>3.0.CO;2-N
301. Freed CR, Greene PE, Breeze RE, Tsai W-Y, DuMouchel W, Kao R, et al. Transplantation of Embryonic Dopamine Neurons for Severe Parkinson’s Disease. N Engl J Med (2001) 344:710–9. doi: 10.1056/NEJM200103083441002
302. Olanow CW, Goetz CG, Kordower JH, Stoessl AJ, Sossi V, Brin MF, et al. A double-blind controlled trial of bilateral fetal nigral transplantation in Parkinson’s disease. Ann Neurol (2003) 54(3):403–14. doi: 10.1002/ana.10720
303. Song H, Stevens CF, Gage FH. Astroglia induce neurogenesis from adult neural stem cells. Nature (2002) 417(6884):39–44. doi: 10.1038/417039a
304. Villa A, Navarro-Galve B, Bueno C, Franco S, Blasco MA, Martinez-Serrano A. Long-term molecular and cellular stability of human neural stem cell lines. Exp Cell Res (2004) 294(2):559–70. doi: 10.1016/j.yexcr.2003.11.025
305. Amit M, Carpenter MK, Inokuma MS, Chiu C-P, Harris CP, Waknitz MA, et al. Clonally Derived Human Embryonic Stem Cell Lines Maintain Pluripotency and Proliferative Potential for Prolonged Periods of Culture. Dev Biol (2000) 227(2):271–8. doi: 10.1006/dbio.2000.9912
306. Kondo T, Funayama M, Tsukita K, Hotta A, Yasuda A, Nori S, et al. Focal Transplantation of Human iPSC-Derived Glial-Rich Neural Progenitors Improves Lifespan of ALS Mice. Stem Cell Rep (2014) 3(2):242–9. doi: 10.1016/j.stemcr.2014.05.017
307. Lepore AC, Rauck B, Dejea C, Pardo AC, Rao MS, Rothstein JD, et al. Focal transplantation–based astrocyte replacement is neuroprotective in a model of motor neuron disease. Nat Neurosci (2008) 11(11):1294–301. doi: 10.1038/nn.2210
308. Sometani A, Kataoka H, Nitta A, Fukumitsu H, Nomoto H, Furukawa S. Transforming growth factor-β1 enhances expression of brain-derived neurotrophic factor and its receptor, TrkB, in neurons cultured from rat cerebral cortex. J Neurosci Res (2001) 66(3):369–76. doi: 10.1002/jnr.1229
309. Johnson-Farley NN, Patel K, Kim D, Cowen DS. Interaction of FGF-2 with IGF-1 and BDNF in Stimulating Akt, ERK, and Neuronal Survival in Hippocampal Cultures. Brain Res (2007) 1154:40–9. doi: 10.1016/j.brainres.2007.04.026
310. Chen P-Y, Qin L, Li G, Tellides G, Simons M. Fibroblast growth factor (FGF) signaling regulates transforming growth factor beta (TGF β )-dependent smooth muscle cell phenotype modulation. Sci Rep (2016) 6(1):1–11. doi: 10.1038/srep33407
311. Bordignon P, Bottoni G, Xu X, Popescu AS, Truan Z, Guenova E, et al. Dualism of FGF and TGF-β Signaling in Heterogeneous Cancer-Associated Fibroblast Activation with ETV1 as a Critical Determinant. Cell Rep (2019) 28(9):2358–72.e6. doi: 10.1016/j.celrep.2019.07.092
312. Fischer ANM, Fuchs E, Mikula M, Huber H, Beug H, Mikulits W. PDGF essentially links TGF- β signaling to nuclear β -catenin accumulation in hepatocellular carcinoma progression. Oncogene (2007) 26(23):3395–405. doi: 10.1038/sj.onc.1210121
313. Hosaka K, Yang Y, Nakamura M, Andersson P, Yang X, Zhang Y, et al. Dual roles of endothelial FGF-2–FGFR1–PDGF-BB and perivascular FGF-2–FGFR2–PDGFRβ signaling pathways in tumor vascular remodeling. Cell Discovery (2018) 4(1):1–14. doi: 10.1038/s41421-017-0002-1
314. McKinnon RD, Matsui T, Dubois-Dalcq M, Aaronsont SA. FGF modulates the PDGF-driven pathway of oligodendrocyte development. Neuron (1990) 5(5):603–14. doi: 10.1016/0896-6273(90)90215-2
Keywords: protective, astrocytes, neuroinflammation, astrogliosis, neurodegeneration, multiple sclerosis, ischemic stroke, Alzheimer’s disease
Citation: Linnerbauer M and Rothhammer V (2020) Protective Functions of Reactive Astrocytes Following Central Nervous System Insult. Front. Immunol. 11:573256. doi: 10.3389/fimmu.2020.573256
Received: 01 July 2020; Accepted: 14 September 2020;
Published: 30 September 2020.
Edited by:
Edgar Meinl, Ludwig Maximilian University of Munich, GermanyReviewed by:
Cinthia Farina, San Raffaele Scientific Institute (IRCCS), ItalyKhalil Sherali Rawji, University of Cambridge, United Kingdom
Copyright © 2020 Linnerbauer and Rothhammer. This is an open-access article distributed under the terms of the Creative Commons Attribution License (CC BY). The use, distribution or reproduction in other forums is permitted, provided the original author(s) and the copyright owner(s) are credited and that the original publication in this journal is cited, in accordance with accepted academic practice. No use, distribution or reproduction is permitted which does not comply with these terms.
*Correspondence: Veit Rothhammer, veit.rothhammer@uk-erlangen.de