ClC-3 regulates the excitability of nociceptive neurons and is involved in inflammatory processes within the spinal sensory pathway
- 1Institute of Biological Information Processing, Molecular and Cellular Physiology (IBI-1), Forschungszentrum Jülich, Jülich, Germany
- 2Medical Imaging Physics, Institute of Neuroscience and Medicine (INM-4), Forschungszentrum Jülich, Jülich, Germany
- 3Institute of Physiology, RWTH Aachen, Aachen, Germany
ClC-3 Cl–/H+ exchangers are expressed in multiple endosomal compartments and likely modify intra-endosomal pH and [Cl–] via the stoichiometrically coupled exchange of two Cl– ions and one H+. We studied pain perception in Clcn3–/– mice and found that ClC-3 not only modifies the electrical activity of peripheral nociceptors but is also involved in inflammatory processes in the spinal cord. We demonstrate that ClC-3 regulates the number of Nav and Kv ion channels in the plasma membrane of dorsal root ganglion (DRG) neurons and that these changes impair the age-dependent decline in excitability of sensory neurons. To distinguish the role of ClC-3 in Cl–/H+ exchange from its other functions in pain perception, we used mice homozygous for the E281Q ClC-3 point mutation (Clcn3E281Q/E281Q), which completely eliminates transport activity. Since ClC-3 forms heterodimers with ClC-4, we crossed these animals with Clcn4–/– to obtain mice completely lacking in ClC-3-associated endosomal chloride–proton transport. The electrical properties of Clcn3E281Q/E281Q/Clcn4–/– DRG neurons were similar to those of wild-type cells, indicating that the age-dependent adjustment of neuronal excitability is independent of ClC-3 transport activity. Both Clcn3–/– and Clcn3E281Q/E281Q/Clcn4–/– animals exhibited microglial activation in the spinal cord, demonstrating that competent ClC-3 transport is needed to maintain glial cell homeostasis. Our findings illustrate how reduced Cl–/H+ exchange contributes to inflammatory responses and demonstrate a role for ClC-3 in the homeostatic regulation of neuronal excitability beyond its function in endosomal ion balance.
Introduction
CLC-type Cl–/H+ exchangers are expressed in the endoplasmic reticulum, Golgi apparatus, and endosomes/lysosomes, with isoform-specific subcellular distributions (Jentsch and Pusch, 2018; Bose et al., 2021). Their physiological importance has been demonstrated by studies of knockout animal models and by naturally occurring mutations in patients with genetic diseases: genetic ablation or naturally occurring mutations in CLCN3 or CLCN7 causes neurodegeneration in the central nervous system (CNS; Kornak et al., 2001; Stobrawa et al., 2001; Dickerson et al., 2002; Kasper et al., 2005; Duncan et al., 2021). Mutations in the CLCN4 gene are associated with intellectual disability and epilepsy (Veeramah et al., 2013; Hu et al., 2016; Palmer et al., 2018; He et al., 2021b; Guzman et al., 2022), and CLCN6 mutations result in West syndrome and lysosomal storage disease (Poet et al., 2006; He et al., 2021a). Genetic ablation of ClC-6 or downregulation of ClC-3 alters pain sensitivity in mice (Poet et al., 2006; Pang et al., 2016), suggesting a role for Cl–/H+ exchangers in pain regulation. However, the functions of CLC transporters in the peripheral nervous system are insufficiently understood.
ClC-3 was suggested to contribute to endosomal acidification (which is initially set by the proton ATPase) by mediating shunting inward chloride currents (Stobrawa et al., 2001). However, the strong outward rectification of ClC-3 (Guzman et al., 2013; Rohrbough et al., 2018) argues against such a function and instead suggests that Cl–/H+ actively acidifies endosomal compartments by exchanging luminal chloride for protons. However, it is unclear how changes in Cl–/H+ exchange modify cell function and how the genetic ablation of individual transporters results in neurodegeneration of variable severity. Impaired pain sensitivity might be caused by a variety of cellular processes; therefore, studying the mechanisms underlying hyperalgesia in Clcn3–/– mice may provide new insight into the cellular functions of CLC-type Cl–/H+ exchangers.
We found that Clcn3–/– sensory dorsal root ganglion (DRG) cells are hyperexcitable, likely due to altered plasma membrane densities of Na+ and K+ channels (Pang et al., 2016). Moreover, we observed microglia activation within the Clcn3–/– dorsal horn of the spinal cord (DHSC). To distinguish whether these alterations are caused by loss of ClC-3-mediated Cl–/H+ exchange or ClC-3 chaperone function, we used a knock-in mouse model (Clcn3E281Q/E281Q) that expresses transport-incompetent ClC-3 E281Q (Guzman et al., 2013). Since ClC-4 is targeted to recycling endosomes and lysosomes in the form of ClC-3/ClC-4 heterodimers, Clcn3E281Q/E281Q/Clcn4–/– double-mutant (DMut) mice were generated to completely abolish ClC-3-associated Cl–/H+ exchange. In these double mutants, disruption of endosomal ClC-3-associated Cl–/H+ exchange did not change the excitability of DRG neurons, suggesting that ClC-3-mediated Cl–/H+ exchange does not regulate the excitability of nociceptive sensory neurons. However, enhanced microglia activation within the spinal tissue of Clcn3–/– and DMut mice indicates that ClC-3 transport is required to maintain neuroglia homeostasis. Our findings demonstrate how ClC-3 Cl–/H+ exchangers orchestrate two distinct processes within the sensory pathway.
Materials and methods
Animal handling and maintenance
ClC-3 knockout mice (Stobrawa et al., 2001) were kindly provided by Dr. T. Jentsch (Leibniz-Institut für Molekulare Pharmakologie and Max-Delbrück-Centrum für Molekulare Medizin, Berlin, Germany) and maintained in house by breeding heterozygous mice. Heterozygous Clcn3+/E281Q was generated by Cyagen Biosciences (Santa Clara, CA, United States) by site-directed mutagenesis in the C57BL/6 background. Homozygous animals were obtained by mating heterozygous mice and identified by PCR using the KAPA Mouse Genotyping Kit (Kapa Biosystems/Roche, KK-7302, Wilmington, MA, United States), according to the manufacturer’s instruction. All Clcn3–/– mice used in this study showed the same phenotype and identical structural changes previously described by others (Stobrawa et al., 2001; Dickerson et al., 2002; Yoshikawa et al., 2002). The lack of commercially available antibodies (Comini et al., 2022) prevented validation of ClC-3 knockout at the protein level. Clcn4–/– mice were generated by Trans Genic Inc. (Kawasaki, Japan), obtained from Deltagen (San Mateo, USA), and maintained as homozygous animals. To generate double-mutant Clcn3E281Q/E281Q/Clcn4–/– homozygous mice, homozygous Clcn4–/– were crossed with homozygous Clcn3E281Q/E281Q; the subsequent crossing of heterozygous first-generation offspring was used to generate Clcn3E281Q/E281Q/Clcn4–/– (Supplementary Figure 1). All animals were genotyped; PCR protocol and primers for genotyping of all mouse modes are provided in Table 1. All mice were housed with a maximum of five mice in the home cage, with food and water ad libitum under controlled conditions with a 12 h–12 h light–dark cycle, air humidity of 55 ± 10%, and a constant room temperature (RT) of 22°C.
Nociceptive testing
Animals of both sexes were used for all nociceptive behavior test experiments, without obvious sex differences. Homozygous female and male mice were compared with their wild-type (WT) littermates at age 21 ± 2 days (P21) or 60 ± 5 days (P60). Before the start of the behavioral test, each mouse was habituated to the testing room for 30 min in a clean single cage. To prevent learning effects, animals were randomly exposed to different temperatures. Each temperature was measured once per day, with at least 1 h between measurements. Animals were rested in empty cages for at least 2 h at RT between the tail flick and hot plate tests.
All behavioral pain experiments were performed by experimenters blinded to the genotype, recorded, and analyzed afterward. For tail flick tests, tails were immersed in water baths (Julabo, Germany) at different temperatures of 46, 48, or 50°C, while the mouse was loosely restrained in the experimenter’s hand. The time before the tail withdrawal was recorded, and each temperature was evaluated in triplicate with a one-day interval between tests. The hot plate test was performed by placing mice onto the metal surface of a heating plate (Ugo Basile S.R.L., Gemonio, Varese, Italy), which was surrounded by a 20-cm-high transparent Plexiglas cylinder and allowed free movement. For this set of experiments, temperatures of 46, 48, 50, and 52°C were used. We avoided higher temperatures, which are often used in this test (Marics et al., 2014), because of the hyperalgesia phenotype of Clcn3–/–. The video-recorded experiments were carefully analyzed using slow-motion presentations. Since a hyperlocomotion phenotype has been described for Clcn3–/– mice (Stobrawa et al., 2001), the time period until the animals showed discomfort by licking or shaking the paw was manually analyzed rather than using an automated device. If the animals did not show any reaction within 30 s, they were immediately removed to avoid tissue damage (Marics et al., 2014). The response of each mouse to each temperature was evaluated once per day in triplicate, with 1 h between measurements and one-day interval between tests. After injection of 5 μl 0.5% (v/v) formalin solution into the right hind paw with a Hamilton microliter syringe (Merck KGaA, Darmstadt, Germany), individual mice were placed into a transparent box (19 cm × 19 cm × 11 cm) with three mirrored walls. The number of paw flinches per minute was recorded and analyzed afterward. The number of flinches was manually counted using slow-motion videos. Measurements were repeated every 5 min for a total of 40 min.
Dorsal root ganglion neuron culture
Dorsal root ganglion neurons were cultured according to a previous protocol with slight modifications (Bost et al., 2017). Dorsal roots were dissected from P21 or P60 animals and cleaned from connective tissue and fibers in ice-cold Locke’s solution (in mM: 154 NaCl, 5.6 KCl, 3.6 NaHCO3, 5 HEPES [4-(2-hydroxyethyl)-1-piperazineethanesulfonic acid), 6 glucose, adjusted to pH 7.3 with NaOH]. After treatment with 200 μl TrypLE Express Enzyme (Cat. 12604013, Gibco, Grand Island, NY, United States) for 5 min at RT, ganglia were immediately transferred into a tube containing 1 ml Neurobasal-A medium (Cat. 10888022, Gibco, Grand Island, NY, United States), 20 μl freshly thawed Liberase (DH Research Grade, Cat. 5401054001, Roche Diagnostics Deutschland GmbH, Mannheim, Germany), and collagenase to a final concentration of 2.3 units/ml and incubated in a water bath at 37°C. Neurons were dissociated using a three-step process: a 7-min incubation, followed by pipetting 10 times with a 1-ml pipette; a 5-min incubation, followed by pipetting 10 times; and a final 5-min incubation, followed by pipetting 15–17 times. After adding 200 μl fetal bovine serum (Cat. 10270-106, Gibco, Grand Island, NY, United States) and incubating at 37°C for 3 min with gentle agitation, cells were sedimented at 400 × g for 4 min. The cell pellet was then washed with 700 μl Dulbecco’s phosphate-buffered saline (PBS; Cat. 14190094, Gibco, Grand Island, NY, United States). After re-pelleting (400 × g for 4 min), freshly prepared NBA-enriched culture medium containing 1% B-27 supplement (Cat. 17504044, Gibco, Grand Island, NY, United States), 1% GlutaMAX (Cat. 35050061, Gibco, Grand Island, NY, United States), 0.4% penicillin/streptomycin (Cat. 15070-063, Gibco, Grand Island, NY, United States), and 5% fetal bovine serum in neurobasal-A medium was added to the cells. The neuronal cell suspension was diluted in 3.5-ml-enriched NBA medium, and approximately, 500 μl cell suspension was seeded onto poly-D-lysine-coated coverslips and cultured at 37°C with 5% CO2 and 90% humidity for 1–5 days. At 24 h after plating, a cocktail of uridine and floxuridine thymidylate synthase inhibitors were added to a final concentration of 40 and 100 mM, respectively; 12 h later, the culture medium was replaced with an enriched NBA medium without inhibitors.
Electrophysiological experiments
Action potentials (APs) were measured using whole-cell recordings in the current-clamp mode under physiological saline solutions, adapted from a published method (Liu et al., 2010; Hoerauf et al., 2015): the bath solution (330 mOsm/kg, adjusted to pH 7.4 with NaOH) contained (in mM) 130 NaCl, 4 KCl, 1 MgCl2, 2 CaCl2, 10 HEPES, and 48 D-glucose. The pipette solution (310 mOsm/kg, adjusted to pH 7.4 with NaOH) contained (in mM) 135 K-gluconate, 7 NaCl, 2 MgCl2, 2 Na-ATP, 0.3 Na2-GTP, 10 HEPES, and 0.2 EGTA. The passive and active properties of the cells were obtained from the voltage responses to 1 s rectangular current pulse injections, with a pre- and post-pulse period of 500 ms. Resting membrane potentials (RMPs) were recorded a few minutes after establishing the whole-cell configuration. Neurons were visualized using an Andor’s Neo 5.5 sCMOs camera attached to the microscope, which is regularly calibrated with a calibration slide. Only neurons with a diameter below 23 μm were used for electrophysiology. Only neurons with a stable RMP between –60 mV and –75 mV and with a series resistance of <12 MΩ were included in the analysis. Input resistance (Rin) was calculated as the slope of the relationship between the voltage response to a current injection within a range from –60 to 20 pA before the first AP. AP thresholds were obtained from the first derivative of the voltage response (Lazarus and Huang, 2011) and AP half-widths from the difference between the rising and decaying phase times at the half-maximum amplitude of the AP. The after-hyperpolarization amplitude (AHP) is defined as the difference between the AP threshold and the minimum voltage response after the peak maximum. All AP properties were analyzed from the first-ever AP using a custom-written Igor-based macro (Igor Pro 7.01 software, WaveMetrics; kindly provided by Dr. Karlijn van Aerde and Dr. Dirk Feldmeyer, INM-10, Forschungszentrum Jülich).
An index to evaluate the intrinsic excitability was used to estimate the excitability of the neuron, as previously described (Lazarus and Huang, 2011): a lower excitability index (EI) indicates a more excitable cell, and a higher EI a less excitable cell (Lazarus and Huang, 2011). The EI is calculated using properties, such as RMP, AP threshold, and Rin, as follows:
Sodium and potassium currents were measured using whole-cell patch-clamp recordings in the voltage-clamp mode. To reduce space-clamp errors, healthy DRG neurons with no obvious processes were used within the first 24 h after plating. For sodium currents, the bath solution contained (in mM) 20 NaCl, 105 choline-Cl, 3 KCl, 1 MgCl2, 1 CaCl2, 10 HEPES, 10 D-glucose, 20 tetraethylammonium chloride, 0.1 CdCl2, and 3 4-aminopyridine (4-AP) at 305 mOsm/kg and pH 7.4 (adjusted with choline-OH), and the pipette solution contained (in mM) 7 NaCl, 105 CsF, 10 EGTA, 10 HEPES, and 50 D-glucose at 300 mOsm/kg and pH 7.4 (adjusted with CsOH). To distinguish tetrodotoxin-sensitive (TTX-S) and TTX-resistant (TTX-R) sodium channels, TTX was applied to a final concentration of 300 nM via a perfusion pipette, and current recordings were compared before and after the application of TTX (Meents and Lampert, 2016; Fischer et al., 2017). For potassium currents, the bath solution contained (in mM) 150 choline-Cl, 5 KCl, 1 MgCl2, 2 CaCl2, 10 HEPES, 1 CdCl2, and 10 D-glucose at 320 mOsm/kg and pH 7.4 (adjusted with choline-OH), and the pipette solution contained (in mM) 120 K-gluconate, 20 KCl, 2 MgCl2, 1 CaCl2, 10 EGTA, 10 HEPES, 5 Mg-ATP, and 0.3 Na2-GTP at 315 mOsm/kg and pH 7.4 (adjusted with NaOH). To block fast-inactivating potassium currents, 5 mM 4-AP was added to the bath solution. In all cases, the osmolality was adjusted with D-glucose and measured with a freezing point osmometer (Osmomat 3000 basic, Gonotec).
Na+ currents were elicited by applying 50-ms test pulses (–100 mV to +40 mV in 5 mV increment every 3 s) from a holding potential of –100 mV. Current inactivation was studied with 500-ms test pulses (–100 mV to +30 mV in 5 mV increment, followed by a 50 ms step at –10 mV) every 3 s from a holding potential of –100 mV. K+ currents were elicited by applying 100 ms test pulses (–80 mV to +60 mV in 10 mV increment every 3 s) from a holding potential of –80 mV. Inward-rectifier K channels (IRK) currents were studied during 200-ms test pulses (0 mV to –160 mV in 10 mV decrement every 3 s) from a holding potential of –50 mV.
Recordings were filtered at 10 kHz and sampled at 100 kHz using an EPC10 double patch amplifier, controlled by PatchMaster (HEKA Elektronik). Borosilicate pipettes (GC150F-10, Harvard Apparatus, United States) were pulled with a resistance of 1.9–2.9 MΩ and coated with a thin layer of wax to reduce the capacitance. Cell capacitance (Cm) and series resistance (Rs) were compensated, and P/4 leak subtraction with a baseline of –80 mV (for K+ currents) and −100 mV (for Na+ currents) was used to correct linear current components. Peak currents for sodium and mean steady-state currents were plotted against the test voltage to obtain current–voltage relationships. Activation curves for sodium currents were derived by plotting normalized GNa as a function of test potential and fitted with the Boltzmann equation (Meents and Lampert, 2016).
Immunohistochemistry
Dissected spinal cord, dorsal roots, and hippocampus were fixed with 4% PFA for 45 min and retinal tissues for 20 min. All fixed tissues were immersed in a 10% sucrose solution for 1 h at RT and incubated overnight in 30% sucrose at 4°C. After cryoprotection, samples were embedded in optimal cutting temperature compound. Tissue sections (20 μm for the spinal cord, dorsal roots, and retina; 100 μm for the hippocampus) were cut using a cryostat, mounted onto single slides, dried for 5 min at RT, and stored at –20°C until use. To unmask antigens and epitopes (for all antibodies except anti-recoverin and anti-rhodopsin antibodies), sections were treated with sodium citrate buffer (10 mM sodium citrate containing 0.05% Tween 20, pH 6.0 adjusted with NaOH) at 80°C for 15 min and then blocked in PBS containing 0.5% Triton X-100, 10% fetal bovine serum, and 1% bovine serum albumin for 2 h at RT. Samples were incubated with primary antibody (Table 2) diluted in blocking solution for 72 h at 4°C (RT for retinal sections). Slides were then washed five times for 10 min each with 0.1 M phosphate buffer (PB; 100 mM Na2HPO4 and 100 mM NH2PO4, adjusted to pH 7.2 with NaOH) and incubated with secondary antibody (Table 2) diluted in blocking solution for 1.5 h at RT in a dark humidified chamber. Finally, slides were washed five times with PB and mounted with Aqua-Poly/Mount on a glass coverslip. Slides were stored overnight at RT until completely dry and stored at –20°C until analysis.
Confocal microscopy and image analysis
Optical images were acquired on a confocal microscope (TCS SP5 II, Leica Microsystems, Germany) using a 20 × /0.70 or 63 × /1.32–0.6 oil immersion objective and digitalized at a resolution of 1024 × 1024 pixels, 200 Hz velocity, and 6-line average in sequential scanning mode. For large tissue imaging, a tile-scan procedure was applied with a 10% stitching threshold. Identical laser intensities and digital gains were used when comparing samples from different phenotypes. Images were processed with FIJI Image J v.1.53c (Wayne Rasband, National Institutes of Health, United States; Schindelin et al., 2012; Rueden et al., 2017), and a self-made pipeline in CellProfiler™ v.3.1.9 cell image analysis software (Broad Institute, Cambridge, MA, United States; Lamprecht et al., 2007; McQuin et al., 2018) was used for automated analysis of cellular fluorescence, area, and shape and number of events. Confocal immunofluorescence images were exported in color and eight-bit grays, and region of interest (ROI) was defined in dorsal horn layers I to IV according to the Allen Brain Atlas of the Mouse Spinal Cord (Lein et al., 2007). Using the point-and-click graphical user interface (GUI), we created a sequential series of modules for image and object processing function. For neuronal counting, “Neurons” for the NeuN staining and “nuclei” for the To-Pro-3 were applied using a global or adaptive threshold strategy. Both objects were delimited using OTSU image processing with either two- or three-class thresholding with a threshold correction factor of 1.0 and a minimum lower bound of 0.2 (pixels below this number were not considered). Neurons and nuclei were identified using a shape method that used peak brightness to identify like-rounded objects. The same approach was used to identify the processes of the astrocytes, microglia, and chemokine ligand (CCL2) staining. The percentage of the area covered by each channel was obtained by dividing it by the area of the ROI. This protocol was standardized with pictures from all phenotypes, and then a high-throughput analysis was done for the whole data set. Although the pipeline was helpful for quantification in the dorsal horn of the spinal cord, counting the DRG neurons was only possible manually using ImageJ.
Western blotting
Tissue samples from at least two animals were homogenized in ice-cold homogenizing buffer containing 2 mM EDTA pH 8.0, 1 mM cOmplete™ (Cat. 5056489001, COEDTAF-RO, Roche, Mannheim, Germany), and protease inhibitor cocktail mPIC, 1:500 dilution (Cat. P8340, Sigma Aldrich- Merck KGaA, Darmstadt, Germany) and sedimented at 100,000 × g for 15 min at 4°C. Pellets were resuspended in solubilization buffer (1% SDS, 10 mM NaPOi (phosphate buffer composed of 92.2 mmol Na2HPO4 and 6.8 mmol NaH2PO4), and 1 mM cOmplete™) and sedimented at 15000 × g for 10 min. Samples were heated at 95°C for 5 min with a 4× loading buffer (200 mM Tris-Cl, pH 6.8, 8% SDS, 4% 2-mercaptoethanol, 50% glycerin, and 0.04% bromophenol blue), and proteins were resolved by polyacrylamide gel electrophoresis. After transfer to PVDF membrane using the semi-dry transfer technique, membranes were dried overnight, blocked for 2 h in 5% nonfat milk solution in PBS containing 0.05% Tween-20 (PBS-T) or in PBS-T containing 3% bovine serum albumin, and incubated overnight at 4°C with primary antibody (anti-rabbit Nav1.8 sodium channel, or mouse anti-α-tubulin; Table 2). Membranes were washed with PBS-T and incubated for 1 h with goat-α-rabbit IgG-horseradish peroxidase (HRP) secondary antibody (Table 2). Membranes were exposed to an equal amount of enhanced chemiluminescent Super Signal™ West Pico PLUS (Cat. 34579, Thermo Scientific, Waltham, MA, United States) substrate, and the exposure time was adjusted depending on protein abundance (1–10 min) to maximize band visibility and minimize background.
Real-time PCR
Total RNA was extracted from freshly dissected DRG neurons from two-month-old mice using the TRIzol™ (Cat. 15596026, Invitrogen, Carlsbad, CA, United States)-chloroform method, and 1 μg total RNA was converted into cDNA using One-Step SuperScript™ III® reverse transcriptase (Cat. 12574018, Invitrogen, Carlsbad, CA, United States) in a Gradient Thermocycler (Biometra, Göttingen, Germany). The master mix contained 12.5 μl 2× reaction mix (containing 0.4 mM each dNTP and 3.2 mM MgSO4), 1 μl enzyme, 0.5 μl 10 mM sense/antisense primers (specific for each isoform), 1 μg template RNA, and RNA-free water to a final volume of 25 μl. cDNA was synthesized by incubation for 30 min at 55°C, followed by 2 min at 94°C. PCR conditions were 40 cycles of 15 s at 94°C (denaturation), 30 s at 55–65°C (annealing), and 5 min at 68°C for the extension, with a final step of 1 min at 68°C. The reference gene was 18S RNA. Primers to identify the ClC isoforms are listed in Table 3. PCR products were assessed by 1% agarose gel electrophoresis [with SYBR™ safe (Cat. S33102, Invitrogen, Carlsbad, CA, United States) staining] at 120 V for 35 min. PCR band size was determined using a GeneRuler 100 bp DNA Ladder (Cat. SM0322, Thermo Scientific, Waltham, MA, United States).
Data analysis
Statistical analysis was performed using OriginPro version 2018b (OriginLab Corporation, Northampton, MA, United States) and Microsoft Excel (Microsoft 365). Data are presented as the mean ± standard error of the mean (SEM) and plotted as boxplots (with boxes indicating the upper and the lower quartiles and whiskers the upper and lower 90 percentiles). The non-paired two-tailed t-test or the Mann–Whitney test was used to compare two groups, depending on whether the data followed a normal distribution. For comparing more than two groups, one-way ANOVA was used for normally distributed data without significant variance inhomogeneity. For more than two factors or independent variables, and one dependent variable, two- or three-way ANOVA was used. If F-value was statistically significant (p < 0.05), a Tukey post hoc test was conducted.
Each data sample was collected randomly and considered independent. We used the Shapiro–Wilk test to evaluate if the data followed a normal distribution and Levene’s test for assessing the homogeneity of variances. Data that did not follow a normal distribution were normalized. If normalization was not possible or variances were not homogenous, a non-parametric test (Mann–Whitney or Kruskal–Wallis ANOVA test) was chosen. Grubb’s test was used to test for outliers. The n value provides the number of cells (or measurement), and N, the number of animals; both values are given in the figure legends. Differences with p-values < 0.05 were considered significant. Asterisk (*) was used to represent differences between groups and (‡) when also animals were compared (*‡p < 0.05, **‡‡p < 0.01; ***‡‡‡p < 0.001).
Results
Genetic ablation of ClC-3 leads to hyperalgesia
Clcn3–/– mice suffer from neurodegeneration in the hippocampus and the retina, with loss of neurons beginning at P21 and completed at P60 (Stobrawa et al., 2001). Since neurodegeneration might affect pain sensitivity, we evaluated pain sensitivity before and after neuronal loss at P21 (young) and P60 (adult). At both developmental stages, we found significantly shorter paw withdrawal latencies to heat stimuli in Clcn3–/– mice than in WT mice (Figures 1A,D). The tail flick test confirmed that Clcn3–/– mice are more sensitive to noxious thermal stimuli than the WT animals (Figures 1B,E). This phenotype was consistently observed for all temperatures evaluated (Supplementary Table 1). In contrast to our results, a previous report did not identify differences in thermal pain perception between Clcn3–/– and WT mice (Pang et al., 2016). Pang et al. (2016) used an automated hot plate device that measured the latency of paw licking, rearing, and jumping in triplicate at 15-min intervals. Repeated pain exposure may have modified reaction latencies in these experiments (Suaudeau et al., 2005) and may have masked the differences in response to noxious temperatures we observed between WT and Clcn3–/– (Figure 1). We, therefore, measured the effects of each temperature only once per day with at least 1 h between measurements.
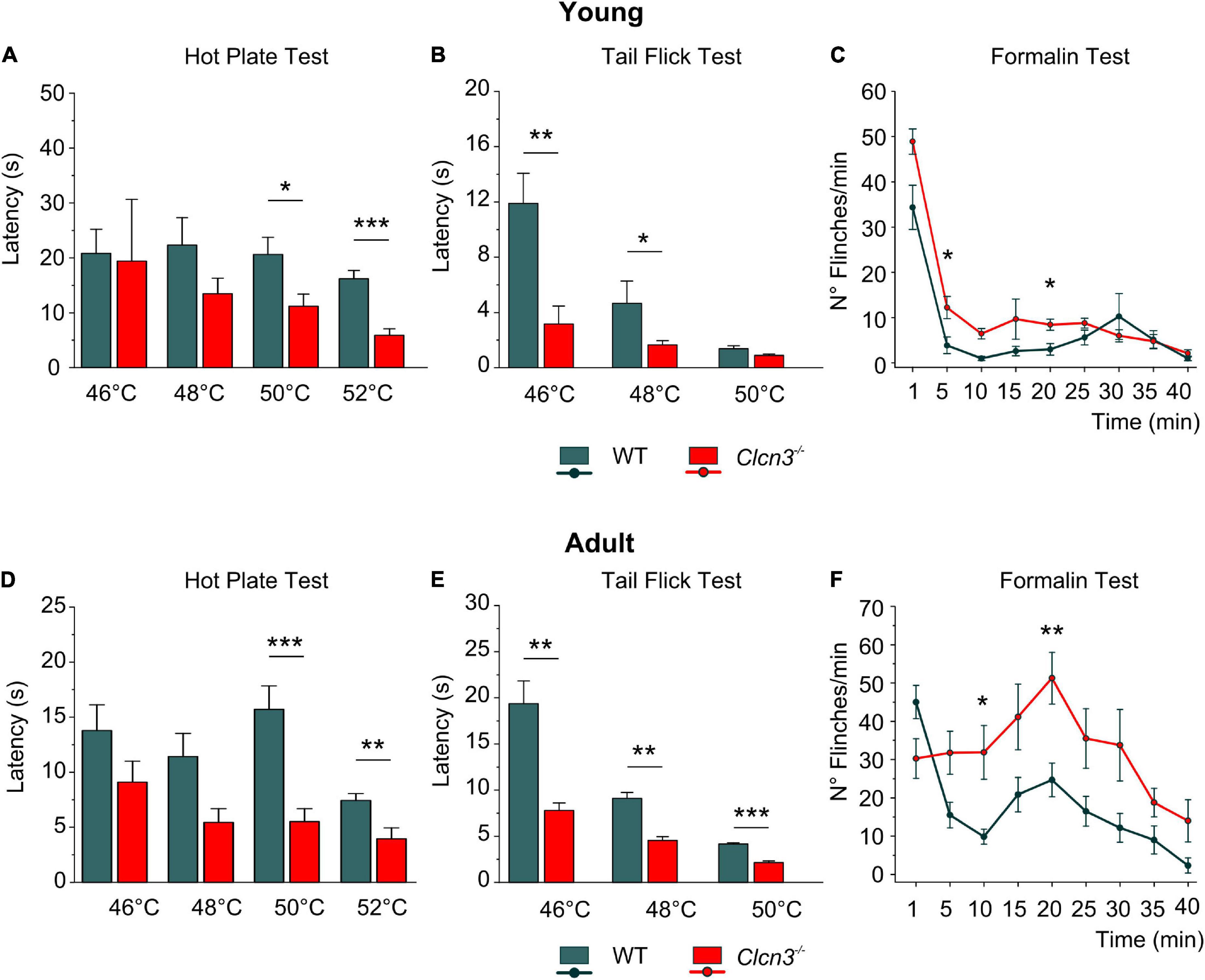
Figure 1. Acute pain experiments in young P21 (A–C) and adult P60 (D–F) mice show increased thermal sensitivity and lower latencies in mutants compared with wild type (WT) animals. (A) Hot plate test, P21; WT (n = 7); Clcn3–/– (n = 8). (B) Tail flick test, P21; WT (n = 8); Clcn3–/– (n = 9). (C) Formalin test, P21; WT (n = 8); Clcn3–/– (n = 9). (D) Hot plate test at different temperatures, P60; WT (n = 11); Clcn3–/– (n = 13). (E) Tail flick test, P60; WT (n = 8); Clcn3–/– (n = 9). (F) Formalin test, P60; WT (n = 6); Clcn3–/– (n = 8). Statistical significance levels are *p < 0.05, **p < 0.01, ***p < 0.001. Clcn3–/– versus WT; n represents the number of animals. Two-way ANOVA or three-way ANOVA analyses were used to detect interactions between variables, and Tukey post hoc test for pairwise comparisons. Data are presented as the mean ± SEM.
Subcutaneous injections of formalin (0.5%) into the hind paw trigger a biphasic pain reaction, comprising an early phase (1–5 min post-injection; Figures 1C,F) due to direct activation of peripheral nociceptors and an inflammatory late-phase reaction (10 min post-injection) associated with persistent pain signals at the supraspinal level (Taylor et al., 1995; Abbadie et al., 1997). Young Clcn3–/– were more sensitive to noxious stimuli at both phases, with significantly more flinches per min in the mutant than in WT animals (Figures 1C; Supplementary Table 2). However, in the late inflammatory phase, only adult Clcn3–/– mice showed more severe reactions than WT (Figure 1F; Supplementary Table 2), suggesting that lack of ClC-3 alters behavioral pain perception at all ages.
Ablation of ClC-3 changes the excitability of sensory dorsal root ganglion neurons
We next examined the electrical properties of sensory neurons from young and adult WT and Clcn3–/– mice using current-clamp recordings. Similar numbers of APs were recorded in DRG neurons from young WT and Clcn3–/– upon injection of depolarizing currents (Figures 2A,B). However, although the firing rates decreased in older WT mice, this developmental change was not observed in mutant mice (Figures 2A,B). The rheobase current (i.e., the minimum current amplitude required to elicit an AP) was reduced in Clcn3–/– adult neurons (Figure 2D), with membrane input resistances significantly higher in both young and adult Clcn3–/– animals (Figures 2C,E). AP thresholds and after-hyperpolarization amplitudes (AHP) were slightly shifted toward more depolarizing potentials in Clcn3–/– mice compared with WT mice (Figure 2F; Supplementary Figure 2), but AP amplitudes and RMPs were unchanged (Figures 2G,H). We also compared the first, second, third, and fourth APs for WT and mutant adult cells and found that AP thresholds, amplitudes, and AHP were not different (Table 4).
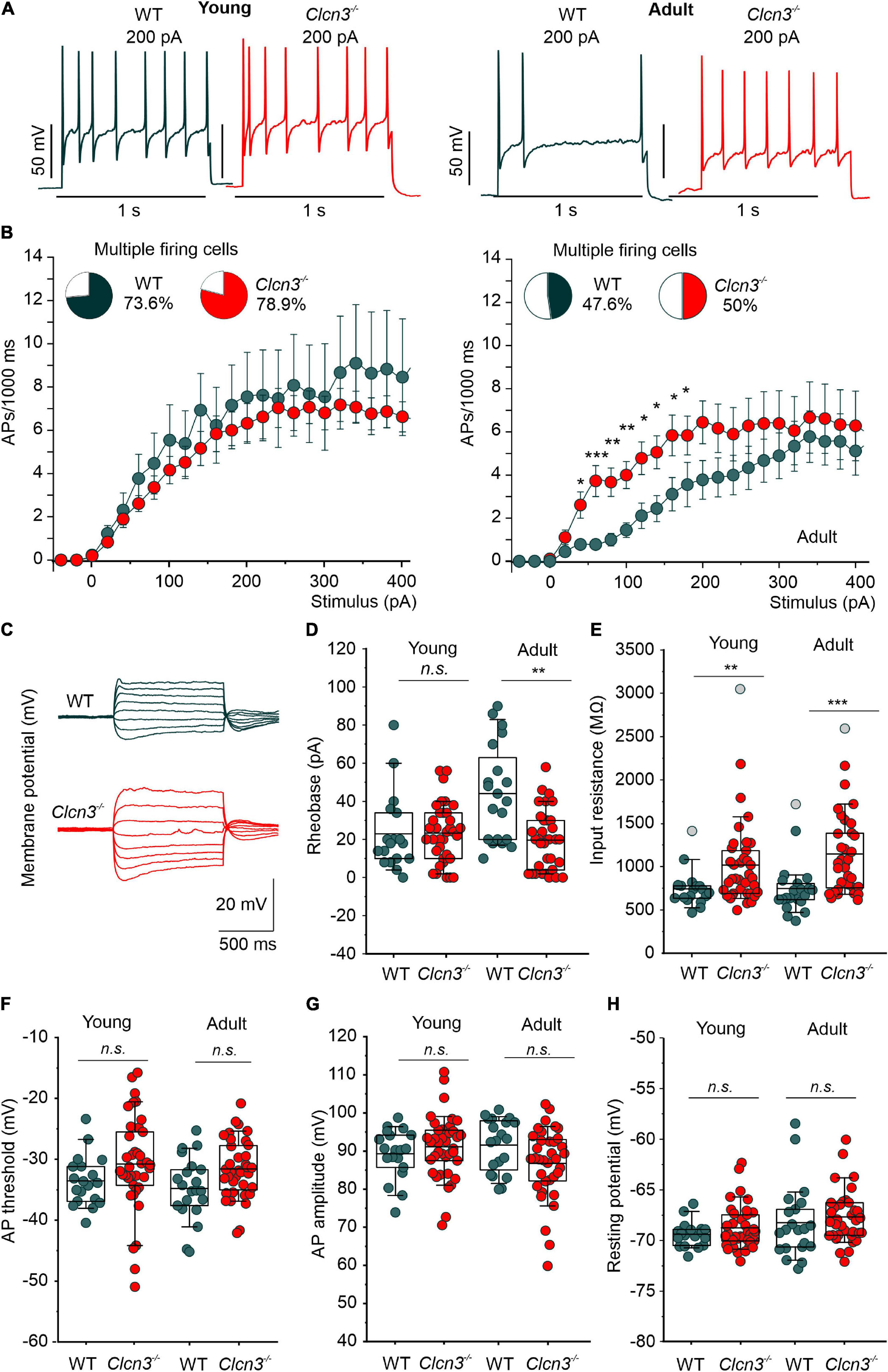
Figure 2. Change in excitability of dorsal root ganglion (DRG) neurons from young and adult Clcn3–/– animals. (A) Representative traces of action potentials (APs) elicited by a current injection of 200 pA in young (left) and adult (right) wild type (WT) (green) and Clcn3–/– (red) neurons. (B) Average firing frequencies from young and adult Clcn3–/– and WT dorsal root ganglion (DRG) neurons. Insets show the percentage of multiple firing neurons for each condition. (C) Representative current responses of control (green) and a Clcn3–/– (red) neuron to hyperpolarizing and depolarizing current pulses. (D) Current threshold necessary to elicit the first action potential (AP; rheobase) in young and adult Clcn3–/– and WT DRG neurons. (E) Average input resistances of DRG neurons from P21 and P60 mice. Symbols in gray are outliers values included in the analysis. (F) AP thresholds, (G) amplitudes, and (H) RMP for young and adult neurons. Data were collected from young WT (n = 19 cells from four animals) and Clcn3–/– (n = 38 cells from four animals) and adult WT (n = 21 cells from seven animals) and Clcn3–/– (n = 36 cells from six animals) cells. Statistical significance levels are *p < 0.05, **p < 0.01, ***p < 0.001, n.s., not significant. A two-way ANOVA or Kruskal–Wallis ANOVA was used to compare the age and phenotype of the two groups. The Tukey post hoc test was used for pairwise comparisons. Data are presented as the mean ± SEM. In boxplots, boxes indicate the upper and lower quartiles, and whiskers the upper and lower 90 percentiles.
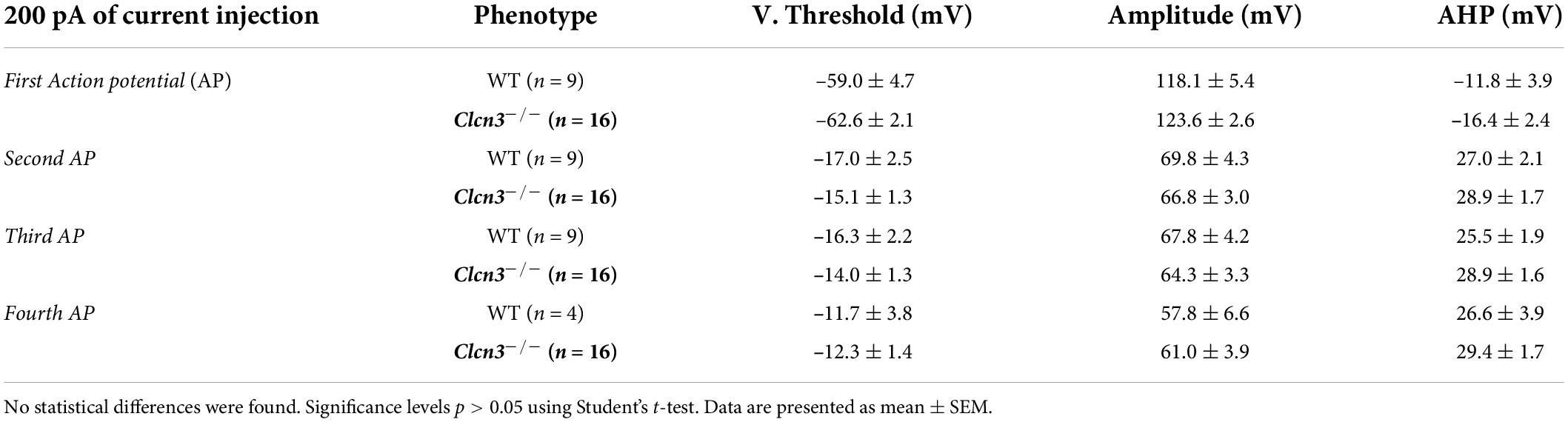
Table 4. Properties of the action potentials (APs) generated in response to 200 pA current injection for 1 s were not different between wild type (WT) and ClCn3–/– adult dorsal root ganglion (DRG) neurons.
We next compared the EI for WT and Clcn3–/– cells. The EI integrates multiple cellular properties (see the equation in the “Materials and methods” section), such as RMP, AP threshold, and the input resistance (Rin) to estimate the intrinsic excitability of a cell (Lazarus and Huang, 2011), with a lower EI indicating increased excitability. Consistent with the age-dependent reduction in neuronal excitability, we obtained lower EIs for DRG neurons from young WT mice compared with adults. However, in DRG neurons from Clcn3–/– mice, EI values did not change with age, resulting in significant differences between adult WT and mutant cells (young DRG, WT 58 ± 4.0 pA vs. Clcn3–/– 47 ± 3.0 pA, Student’s t-test p = 0.05; adult DRG, WT 74 ± 9.0 pA vs. Clcn3–/–, 42 ± 3.0 pA, Mann–Whitney test p = 0.0017). We conclude that ClC-3 transporters contribute to the age-dependent regulation of neuronal excitability in sensory DRG cells.
Clcn3 deletion alters ion channel density in the plasma membrane of dorsal root ganglion neurons
Voltage-gated sodium channels are necessary to initiate and propagate APs, and changes in the expression or function of these channels are associated with various pain disorders (Cummins and Waxman, 1997; Berta et al., 2008; Dib-Hajj et al., 2010; Lampert et al., 2010; Bernal, 2018). Small-diameter DRG neurons express fast-inactivating TTX-sensitive (TTX-S) Nav1.1, Nav1.6, and Nav1.7 and slow-inactivating TTX-resistant (TTX-R) Nav1.8 and Nav1.9 (Rush et al., 2007) channels. In patch-clamp experiments, we measured sodium currents in DRG neurons from Clcn3–/– and WT adults before and during the extracellular application of 300 nM TTX, using the same voltage protocol. Total Na+ current densities were slightly increased in Clcn3–/– DRG neurons (Figures 3A,B), along with a significantly decreased TTX-R component (Figures 3A,C). To determine the TTX-S Na+ current, the TTX-R component was subtracted from the total Na+ current. The TTX-S current did not differ between Clcn3–/– and WT cells (Figures 3A,D). Western blotting showed that Nav1.8 protein levels were not reduced in Clcn3–/– cells (Supplementary Figure 3), indicating that loss of ClC-3 does not affect the de novo synthesis of Nav1.8 channels. We observed minor changes in the voltage dependence of sodium channel activation and inactivation in Clcn3–/– neurons compared with WT neurons (Supplementary Figures 4A–F), probably due to changes in the relative amplitudes of different sodium channels in DRG neurons. Taken together, these observations suggest that ClC-3 regulates the surface membrane insertion of the TTX-R Nav1.8/Nav1.9, but not of the Nav1.1, Nav1.6, or Nav1.7 channels.
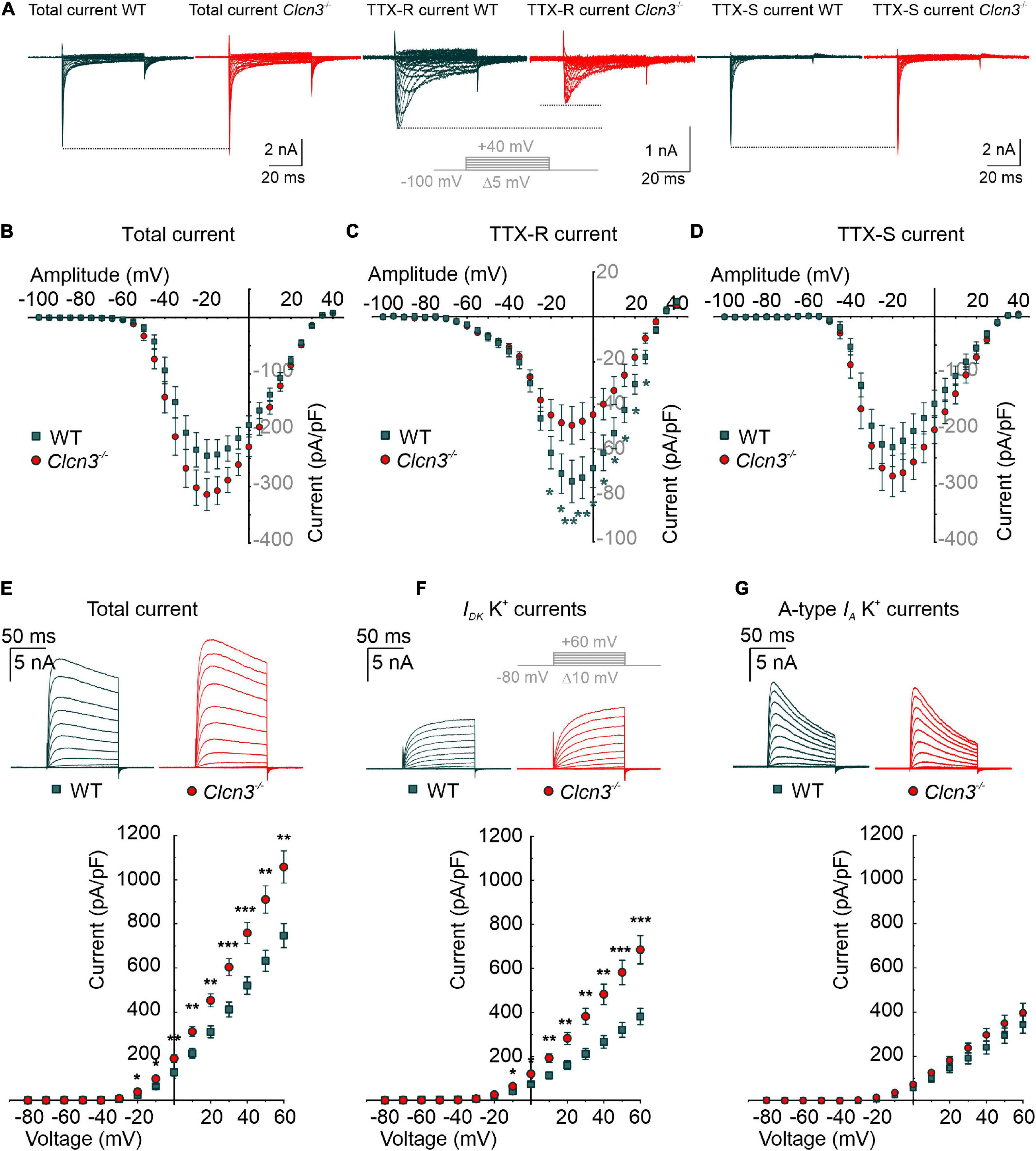
Figure 3. Whole-cell Na+ and K+ currents from cultured wild type (WT) and Clcn3–/– dorsal root ganglion (DRG) adult neurons. (A) Representative traces of Na+ currents before (total Na+ current) and during the application of 300 nM TTX (TTX-R current), and after digital subtraction of the TTX-R current from the total Na+ current (TTX-S current) from WT (green) and Clcn3–/– (red) cells. The inset illustrates the voltage protocol used to elicit Na+ currents during and after TTX application. (B–D) Current–voltage relationships of total (B), TTX-R (C), and TTX-S (D) Na+ current densities from WT (green, n = 23 from five animals) and Clcn3–/– (red, n = 23 from eight animals) DRG neurons. Traces were aligned to the holding current at –100 mV, and dotted lines were set to the maximum pick current of the WT to illustrate changes in the Clcn3–/–. (E–G) Representative recordings of the K+ current (upper panel) and current–voltage relationship (lower panel) were obtained from WT (green, n = 16 from five animals) and Clcn3–/– (red, n = 20 from three animals) DRG neurons. Inset in gray depicts the voltage protocol used to elicit K+ currents during and after 4-AP application. (E) Total K+ current densities are significantly higher in the absence of ClC-3. (F) Slow-inactivating K+ currents obtained from the same neuron showed in E, after the application of 5 mM 4-AP, a compound that blocks fast-inactivating K+ channels, showed a similar phenotype as for the total K+ current. (G) Fast-inactivating K+ currents are obtained by subtracting the slow-inactivating (F) from the total (E) current. Statistical significance levels are *p < 0.05, **p < 0.01, and ***p < 0.001; Student’s t-test. Data are presented as mean ± SEM.
Altered nociception has also been associated with changes in the surface expression and biophysical properties of K+ channels (Duan et al., 2012; Laumet et al., 2015; Conner et al., 2016). In whole-cell recordings, outward-rectifier potassium currents were larger in small-diameter DRG neurons from adult Clcn3–/– mice than in those from adult WT mice (Figure 3E). To identify which types of K+ channels are affected by ClC-3 deletion, we recorded K+ currents before and during the application of 5 mM 4-AP. 4-AP blocks A-type fast-inactivating voltage-gated K+ currents (IA), whereas both slow-inactivating voltage-gated K+ currents (ID) and delayed-rectifier voltage-gated K+ currents (IK) are 4-AP-insensitive (Vydyanathan et al., 2005). ID and IK currents were larger in neurons from adult Clcn3–/– mice compared with adult WT mice (Figure 3F), whereas IA currents did not differ (Figure 3G). DRG neurons also express inward-rectifier potassium channels (Kir2.3 and Kir2.4) (Busserolles et al., 2020) or Kir3.1 (Seitz et al., 2021). Electrophysiological recording of inward-rectifier potassium channels showed small currents of up to 300 pA at -160 mV (Supplementary Figure 4G), with no difference between WT and Clcn3–/– DRG neurons (Supplementary Figure 4H). We conclude that ClC-3 regulates the plasma membrane density of slow-inactivating and delayed-rectifier voltage-gated K+ channels, but not of other K+ channels.
ClC-3 Cl–/H+ transport is not required for the normal excitability of sensory dorsal root ganglion cells
ClC-3 exchanges Cl– for H+ at a fixed stoichiometry of 2:1 and, thus, might modify endosomal [Cl–] or pH (Guzman et al., 2013; Rohrbough et al., 2018). To separate the function of ClC-3-associated Cl–/H+ exchange in regulating neuronal excitability from other ClC-3 functions, we used a knock-in animal model expressing mutant ClC-3 with a neutralizing point mutation in the proton glutamate: E281Q, E339Q, and E312Q in ClC-3a, ClC-3b, and ClC-3c, respectively (Accardi et al., 2005; Guzman et al., 2013; Rohrbough et al., 2018). In each isoform, this mutation abolishes Cl–/H+ exchange but does not affect the ability to heterodimerize with ClC-4 and to target ClC-4 to recycling endosomes or late endosome/lysosomes (Guzman et al., 2013, 2017). Since ClC-4 is also expressed in DRG neurons (Supplementary Figure 5), we generated the double-mutant Clcn3E281Q/E281Q/Clcn4–/– to completely abolish ClC-3-associated Cl–/H+ exchange in all organelles. Clcn4–/– and Clcn3E281Q/E281Q pups are born at the Mendelian ratio, are fertile, and develop normally with no obvious phenotype. In contrast, Clcn3E281Q/E281Q/Clcn4–/– are born at the Mendelian ratio; they show a stronger phenotype than the Clcn3–/–, are smaller, and develop with delay in comparison to littermates.
For DRG neurons isolated from P60 animals, AP frequencies were similar for WT, Clcn3E281Q/E281Q, Clcn4–/–, and Clcn3E281Q/E281Q/Clcn4–/– (Figures 4A,B). All AP parameters tested were indistinguishable between DRG neurons from P60 mutant and WT mice (Figures 4C,D and Supplementary Figure 6). Total potassium currents were similar between Clcn3E281Q/E281Q/Clcn4–/– and WT cells. Furthermore, the separation of A-type fast-inactivating from slow-inactivating voltage-gated K+ channels confirmed the WT-like phenotype of Clcn3E281Q/E281Q/Clcn4–/– neurons, with IA, ID, and IK currents similar to those of WT neurons (Figures 4E–G). These results suggest that ClC-3-associated Cl–/H+ exchange is not required for normal excitability in sensory DRG neurons.
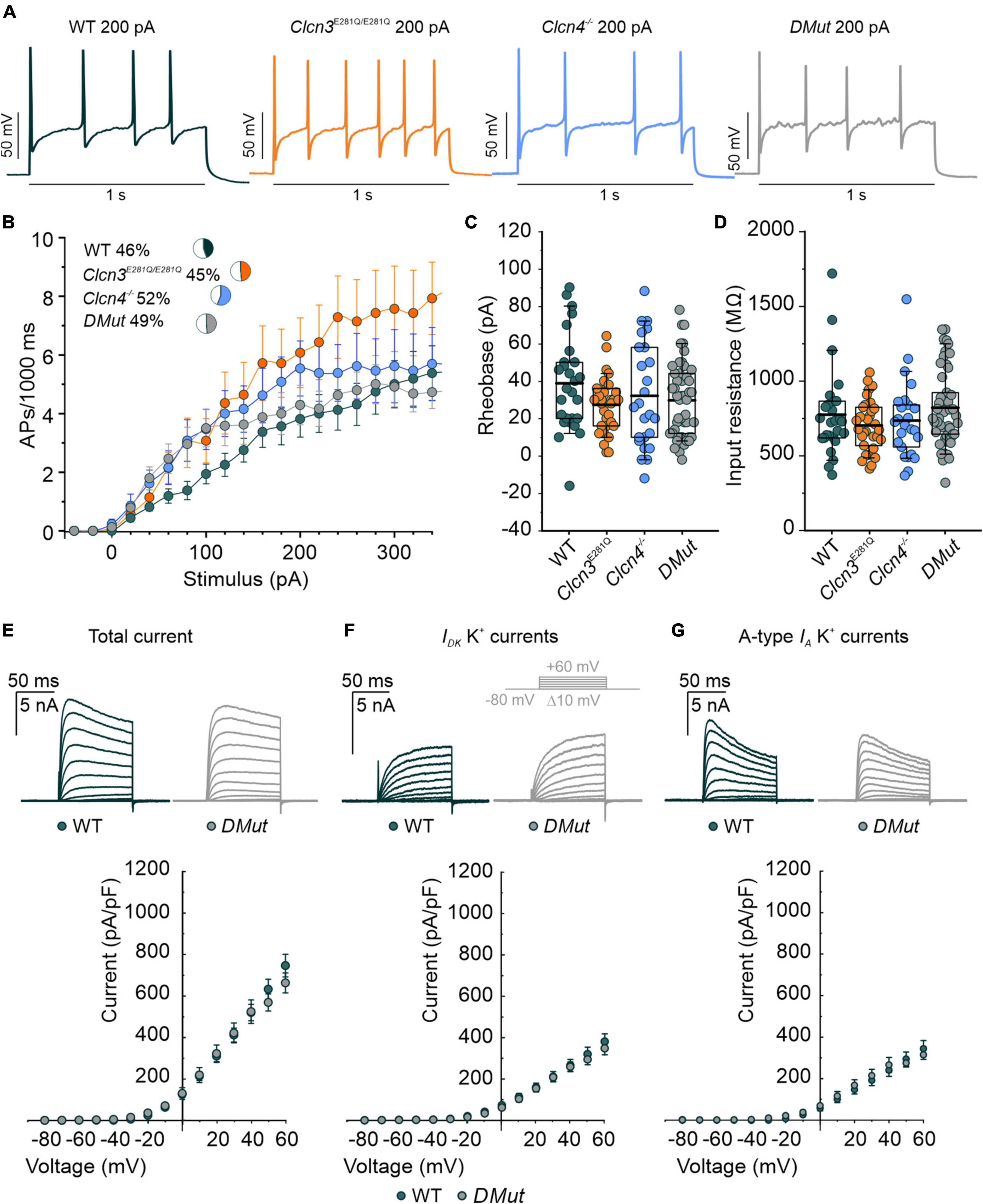
Figure 4. Comparison of the action potential (AP) properties of wild type (WT), Clcn3E281Q/E281Q, Clcn4–/–, and Clcn3E281Q/E281Q/Clcn4–/– (DMut) dorsal root ganglion (DRG) neurons from adult mice. (A) Representative APs elicited by 200 pA current injection in adult WT (green), Clcn3E281Q/E281Q (orange), Clcn4–/– (blue), and DMut (gray) neurons. (B) AP frequencies for all conditions. Insets show the percentage of multiple firing neurons for each condition. (C) Rheobase and (D) input resistance. Data were collected from WT (green, n = 27 cells from three animals), Clcn3E281Q/E281Q (orange, n = 31 cells from three animals), Clcn4–/– (blue, n = 25 cells from three animals), or Clcn3E281Q/E281Q/Clcn4–/–– (gray, n = 49 from seven animals) neurons. (E–G) Representative recordings of the K+ current (upper panel) and current–voltage relationship (lower panel) were obtained from WT (green, n = 16 from five animals) and DMut (gray, n = 10 from three animals) DRG neurons. The inset illustrates the voltage protocol used to elicit K+ currents during and after 4-AP application. Total (E), slow-inactivating (F), and fast-inactivating (G) K+ currents densities are not different between phenotypes. One-way ANOVA or one-way Kruskal–Wallis ANOVA was used for statistical analysis. Data are presented as the mean ± SEM. In boxplots, boxes indicate the upper and lower quartiles, and whiskers the upper and lower 90 percentiles.
Cl–/H+ transport activity regulates microglia activation
Microglia are a specialized population of macrophage-like cells in the CNS that modulate neuronal activity and neuronal excitability in nociceptive pathologies (Vallejo et al., 2010). Immunohistochemical analysis with antibodies against the microglial marker integrin alpha-M or also known as cluster of differentiation molecule 11B (CD11b) and the astrocyte marker glial fibrillary acidic protein (GFAP) (Lan et al., 2017) was conducted to evaluate glial activation in Clcn3–/– from adult mice (Figure 5A, dashed line, and Figure 5Da,b and Supplementary Figure 7). In layers I, II, III, and IV of the DHSC of P60 animals, GFAP levels were increased by about 89% and CD11b levels by 49% in Clcn3–/– compared with WT (Figure 5Da,b). In P21 Clcn3–/– mice, microglia and astrocyte proliferation were increased (Supplementary Figure 8). These results indicate that neuroinflammatory changes are associated with glial activation in the dorsal horn of the Clcn3–/– spinal cord.
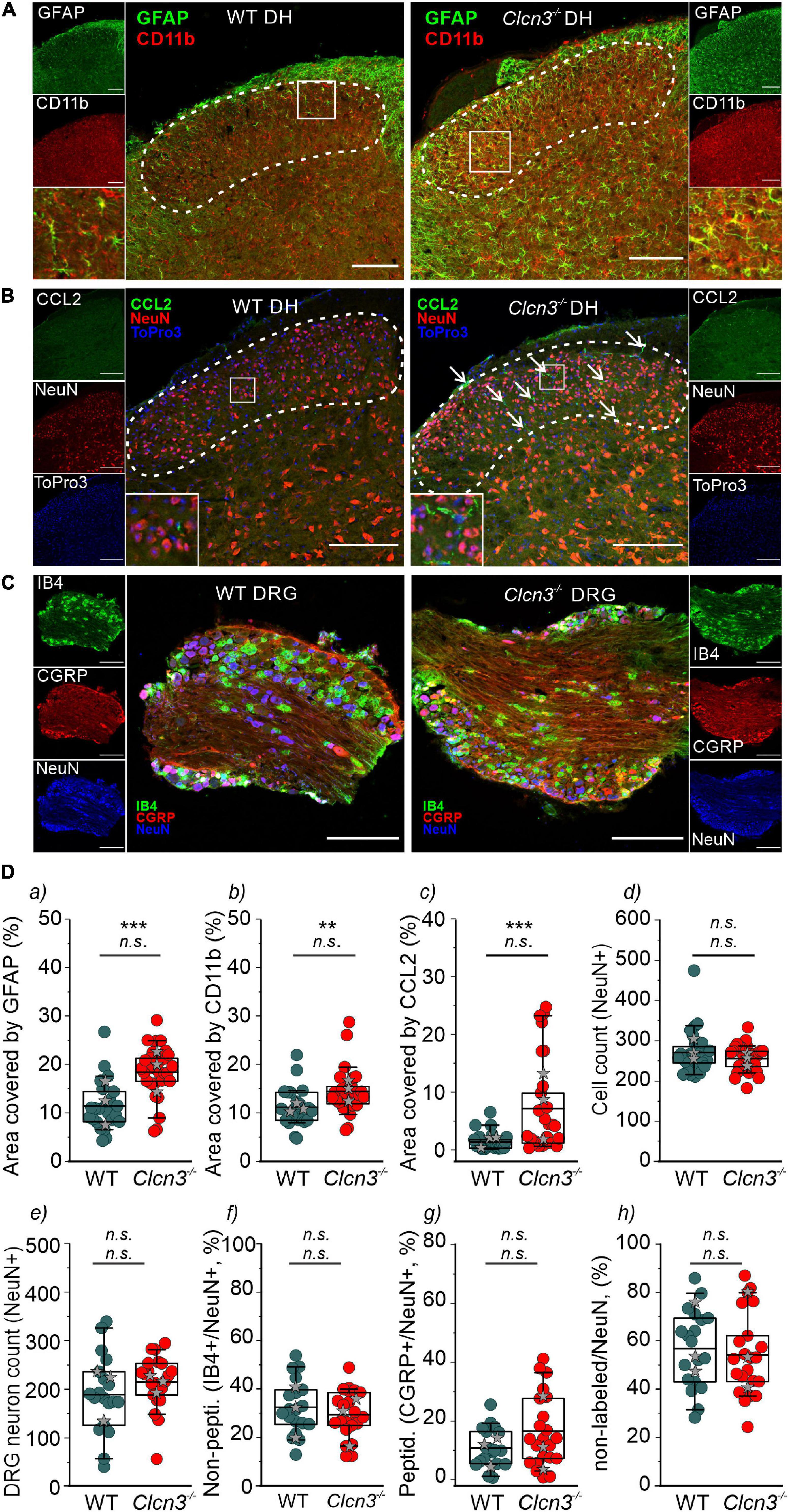
Figure 5. ClC-3 disruption leads to neuroinflammation within the DHSC. (A) glial fibrillary acidic protein (GFAP) and CD11b positivity in the dorsal horn (DH) layers I–IV of wild type (WT) and Clcn3–/– mice. (B) Section of the lumbar spinal cord from WT and Clcn3–/– mice stained for CCL2 (green), NeuN (red), and TO-PRO-3 (blue, nuclei). (C) Representative immunofluorescence confocal images of dorsal root ganglia from WT and Clcn3–/– mice stained for NeuN (blue), IB4 non-peptidergic small-diameter (green), and CGRP peptidergic (red) neurons. (D) (a) Area of glia expressing GFAP, (b) activated microglia expressing CD11b, (c) area of neuronal CCL2 expression, and (d) neuronal count in the dorsal horn from WT and mutant spinal cords. (e–h) Quantitative analysis of the total neuron count within the dorsal root ganglion (DRG). Each dot represents a single measurement, (a–d) WT (n = 29), Clcn3–/– (n = 33); (g, h) WT (n = 23), Clcn3–/– (n = 29). Three or four mice were used per genotype, with at least six slices from the lumbar section of each animal. Sections were 20-μm thick and collected every 100 μm apart. Scale bar: 200 μm. Statistical significance levels are **p < 0.01, ***p < 0.001, n.s., not significant. Clcn3–/– differs from WT using the Student’s t-test (upper symbol), and statistics per animal with the data displayed with gray stars (lower symbol). In boxplots, boxes indicate the upper and lower quartiles, and whiskers the upper and lower 90 percentiles.
Chemokine ligand 2 is a chemotactic factor that attracts immune cells, such as monocytes/macrophages and natural killer cells. Increased CCL2 expression in DRG neurons and the spinal cord has been associated with inflammatory pain and hypersensitivity (Menetski et al., 2007; Illias et al., 2018; Gschwandtner et al., 2019). At P60, fine cellular processes reactive to CCL2 were readily visible and more abundant within the DHSC region in Clcn3–/– than in WT mice (Figure 5B), resulting in larger CCL2-stained areas in the Clcn3–/– dorsal horn (Figure 5Dc). These observations are consistent with increased CCL2 secretion contributing to the development of hyperalgesia in Clcn3–/–. To test for neurodegeneration, dorsal root ganglia or spinal cord sections were stained for the neuronal marker NeuN. This revealed no difference in the total number of neurons between the dorsal root ganglia of P60 WT and mutant animals (Figures 5C,De). Isolectin B4 (IB4) is a marker for non-peptidergic neurons, and calcitonin gene-related peptide (CGRP) is a marker for peptidergic neurons (Priestley, 2009). Staining for IB4 and CGRP revealed more non-peptidergic IB4-positive cells than peptidergic CGRP-positive cells (Figure 5Df,g) in both WT and Clcn3–/– mice, but no differences in the number of cells between WT and mutant tissues. Results were similar for large- and medium-diameter neurons, which are NeuN-positive, but negative for IB4 and CGRP (Figure 5Dh). The DHSC receives nociceptive inputs in layers I and II and non-nociceptive inputs in layers I, IV, and V from small-, medium-, and large-diameter DRG neurons (Stucky, 2007). Similar to the dorsal root ganglia, neuron numbers in layers I–IV of the DHSC were not reduced in Clcn3–/– compared with WT animals (Figure 5Dd). We conclude that genetic ablation of ClC-3 does not lead to neurodegeneration in the dorsal root ganglia or in the dorsal horn of the spinal cord.
To investigate the role of ClC-3-associated Cl–/H+ exchange in these inflammatory processes, we compared GFAP and CD11b levels in WT, Clcn3E281Q/E281Q, Clcn4–/–, and Clcn3E281Q/E281Q/Clcn4–/– mice (Figure 6). Numbers of neuronal cells were similar (Figure 6C), and areas reactive to GFAP or positive for the microglia marker CD11b were significantly increased in all genotypes (Figures 6A,D,E). Moreover, glial cells had more and longer processes in mutants than in WT mice (Figures 6A,B, insets). The CCL2-positive area was ∼30-fold greater in Clcn3E281Q/E281Q, Clcn4–/–, and Clcn3E281Q/E281Q/Clcn4–/– than in WT mice (Figures 6B,F). Overall, these findings demonstrate that fully functional ClC-3 and ClC-4 chloride transporters are required to prevent microglia and astrocyte proliferation.
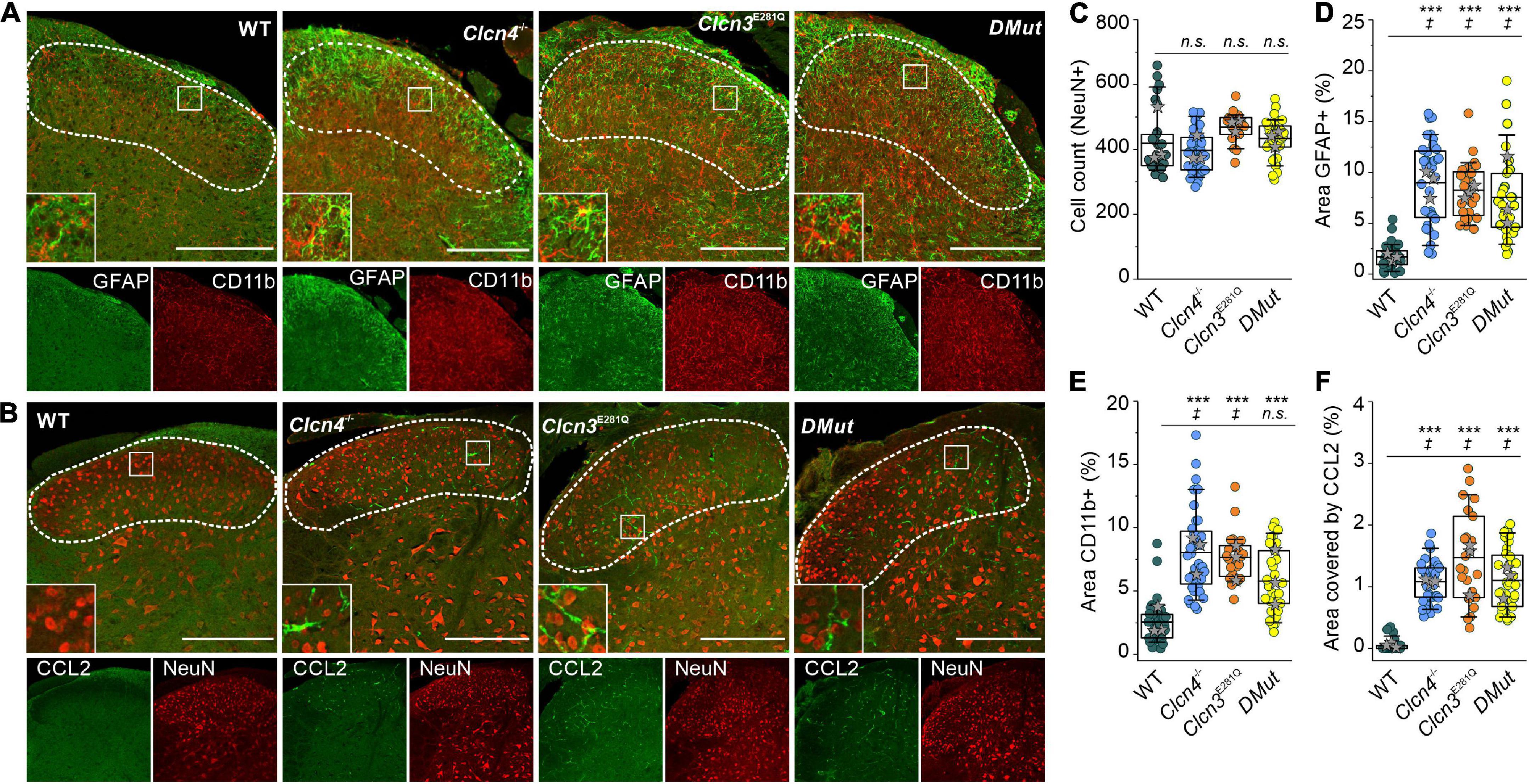
Figure 6. Reduced Cl–/H+ exchange triggers neuroglia activation in the spinal cord. (A) Representative immunofluorescence confocal images of lumbar spinal cord sections from WT, Clcn3E281Q/E281Q, Clcn4–/–, and Clcn3E281Q/E281Q/Clcn4–/– (DMut) mice stained for glial fibrillary acidic protein (GFAP) (green) and CD11b (red). Layers I–IV of the dorsal horn are outlined with a dashed line. Insets show increased GFAP and CD11b reactivity in mutant mice. (B) Lumbar spinal cord sections from mice with the indicated genotypes were stained for CCL2 (green) and NeuN (red). (C) Total cell numbers in the dorsal horn of WT and mutant’s spinal cords. (D) Areas of glia positive for GFAP or to CD11b in E for WT (n = 34), Clcn3E281Q/E281Q (n = 24), Clcn4–/– (n = 36), and Clcn3E281Q/E281Q/Clcn4–/– (DMut; n = 30). (F) Area of CCL2 immunofluorescence in WT (n = 30), Clcn3E281Q/E281Q (n = 23), Clcn4–/– (n = 34), and Clcn3E281Q/E281Q/Clcn4–/– (n = 36). Each dot represents a single measurement. Three or four mice were used per genotype, with at least six slices from the lumbar section of each animal. Sections were 20-μm thick and collected every 100 μm apart. Scale bar: 200 μm. Asterisk (*) was used to represent differences between groups and (‡) when also animals were compared (‡p < 0.05, ***p < 0.001). n.s., not significant; one-way ANOVA (Tukey’s HSD post hoc test). In boxplots, boxes indicate the upper and lower quartiles, and whiskers the upper and lower 90 percentiles.
Neurodegeneration in Clcn3–/–, Clcn3E281Q/E281Q, Clcn4–/–, and Clcn3E281Q/E281Q/Clcn4–/– animals
Hippocampal and retinal neurodegeneration is well established in Clcn3–/– animals (Stobrawa et al., 2001; Dickerson et al., 2002). The lack of neurodegeneration in the DRG or DHSC region (Figure 5) indicates that separate classes of neurons have distinct sensitivities to reduced levels of Cl–/H+ exchange. This finding prompted us to study neurodegeneration in the hippocampus and retina in various animal models with distinct levels of endosomal Cl–/H+ exchange (Figure 7). In agreement with previous studies, ClC-3 ablation resulted in severe neurodegeneration in the hippocampus and retina (Figure 7; Stobrawa et al., 2001; Dickerson et al., 2002). Clcn4–/– mice do not exhibit hippocampal or retinal degeneration (Figure 7). Clcn3E281Q/E281Q animals had normal hippocampal morphology but the reduced thickness of the photoreceptor layer (Figure 7C), indicating that the retina is more sensitive than the hippocampus to reduced levels of ClC-3-associated Cl–/H+ exchange. CA1 and CA2 hippocampal regions and retinal tissue in Clcn3E281Q/E281Q/Clcn4–/– mice were almost completely absent at the age of P21 (Figures 7A,B). These findings highlight the sensitivity of neuronal tissues to reduced Cl–/H+ exchange. Whereas the DHSC is viable in the complete absence of ClC-3-associated transport in Clcn3–/–, the retina is degenerated by the absence of ClC-3-mediated Cl–/H+-exchange even in presence of ClC-4. The hippocampus tolerates reduced Cl–/H+-exchange in Clcn3E281Q/E281Q, but is complete loss in Clcn3E281Q/E281Q/Clcn4–/– and Clcn3–/–.
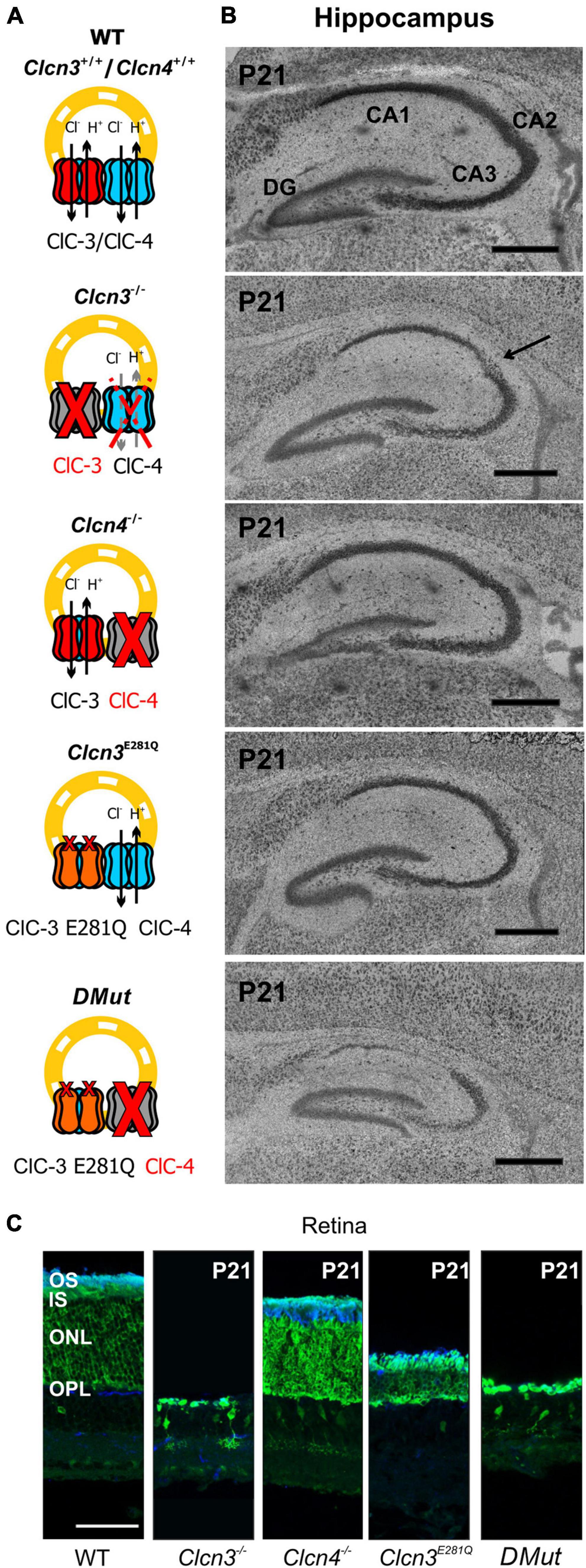
Figure 7. Hippocampal and retinal degeneration in different animal models of Cl–/H+ exchange. (A) Cartoons illustrating heterodimerization between ClC-3 and ClC-4 and the contribution of Cl–/H+ transport function in endosomes for each genotype and (B) representative confocal images of Nissl-stained sagittal (100 μm) section indicating hippocampal formation from 21-day-old WT, Clcn3–/–, Clcn3E281Q/E281Q, Clcn4–/–, and Clcn3E281Q/E281Q/Clcn4–/– (DMut) mice. The arrow indicates neurodegeneration in the CA2 region of the Clcn3–/– hippocampus. (C) Representative immunofluorescence confocal images of retinal sections from different mouse genotypes at P21. Sections were immunostained using an anti-recoverin antibody to indicate the inner segments, somas, and terminals of photoreceptors (green; this antibody also occasionally labels type 2 bipolar cells) and anti-rhodopsin to identify the outer segment of rod cells (blue). OS, outer segment; IS, inner segment; ONL, outer nuclear layer; OPL, outer plexiform layer. Scale bar: 0.5 mm for hippocampal and 50 μm for retinal images.
Discussion
We have demonstrated that genetic ablation of the Cl–/H+ exchanger ClC-3 alters nociception in mice. In Clcn3–/– animals, we observed enhanced electrical activity of peripheral nociceptors and inflammatory processes at the spinal cord. We considered that ClC-3 might affect cellular processes either by changing chemical or electrical gradients at endosomes or by supporting the intracellular trafficking of other proteins via a chaperone-like function. To distinguish between these possibilities, we generated mutant mice (Clcn3E281Q/E281Q) that express transport-incompetent ClC-3. Since E281Q ClC-3 can still heterodimerize with the related transporter ClC-4, the genetic ablation of ClC-4 was also necessary to abolish all ClC-3-associated Cl–/H+ exchanges in recycling endosomes and lysosomes. Clcn3E281Q/E281Q/Clcn4–/– animals had an inflammatory response in the DHSC and early onset hippocampal and retinal degeneration, illustrating the importance of ClC-3 Cl–/H+ transport for neuroglia function and neuronal integrity in supraspinal brain regions. In contrast, the electrical properties of sensory DRG neurons were similar in Clcn3E281Q/E281Q and Clcn3E281Q/E281Q/Clcn4–/– mice, indicating that ClC-3-dependent regulation of neuronal excitability is independent of Cl–/H+ exchange.
ClC-3 ablation impairs age-related adjustment in neuronal excitability, which is thought to result from developmental changes in the expression and/or function of ion channels, such as Na+ and K+ channels (Scott et al., 1988; Wang and Albers, 2009). Specifically, reduced K+ conductances were proposed to reduce excitability in old neurons (Scott et al., 1988). DRG neurons from adult Clcn3–/– (but not Clcn3E281Q/E281Q/Clcn4–/– mice) had increased densities of slow-inactivating and delayed-rectifier voltage-gated K+ currents (Figure 3F). Other current components, such as IA and inward-rectifying voltage-gated K+ currents, were unaffected by ClC-3 ablation. Two-pore domain K+ (K2P) channels help to set and stabilize the RMP (Rasband et al., 2001). Since the RMP was unchanged, we excluded the possibility that the expression or trafficking of K2P channels is altered in Clcn3–/– mice. A greater number of voltage-dependent K+ channels in knockout cells may facilitate AP repolarization and enhance excitability (Scott et al., 1988). We found a reduced density of TTX-R Na+ currents mediated by Nav1.8 and/or Nav1.9 in DRG neurons from adult Clcn3–/– mice (Figure 3C), but no significant change in TTX-S currents (Figure 3D), which are predominately mediated by Nav1.7 (Chen et al., 2018). Modeling studies have demonstrated that the excitability of peripheral neurons depends on the ratio of Nav1.7 to Nav1.8 (Petersson et al., 2014; Tigerholm et al., 2015); thus, the observed changes in sodium currents can explain the observed hyperexcitability of Clcn3–/– DRG. TTX-R currents play a dominant role in all phases of the AP (Blair and Bean, 2002) and are believed to adjust the AP voltage threshold in DRG neurons (Bennett et al., 2019). Our results indicate that ClC-3 contributes to the homeostatic regulation of neuronal excitability by controlling the number of ion channels, such as Na+ and K+ channels in the surface membrane. Since these changes were not found in Clcn3E281Q/E281Q/Clcn4–/– mice, we conclude that the regulatory mechanism is not mediated by Cl–/H+ exchange activity but instead by a chaperone function of ClC-3.
Formalin injection induces a biphasic pain reaction (Figure 1). During the early phase, activation of C-type nociceptors and the release of mediators, such as ATP, glutamate, kinins, histamine, serotonin, cytokines, and tropic factors, promote peripheral sensitization (Hunskaar and Hole, 1987; Amaya et al., 2013). The second phase is dominated by inflammatory responses in the DHSC (Hunskaar and Hole, 1987; Tjølsen et al., 1992). Young and adult Clcn3–/– mice were more sensitive to formalin application. This enhanced behavioral pain response during the second phase suggests that a central sensitization within the dorsal horn changes the elicited sensory response and releases pro-inflammatory mediators to the spinal cord in Clcn3–/– (Ren and Dubner, 2008; Vallejo et al., 2010; Mika et al., 2013). We found increased numbers of activated astrocytes and microglia within the spinal cord of Clcn3–/– mice at P21 and P60 (Supplementary Figure 8; Figure 5). The identification of reactive microglia in young and adult Clcn3–/– and in adult Clcn4–/–, Clcn3E281Q/E281Q, and Clcn3E281Q/E281Q/Clcn4–/– mice (Figure 5; and Figure 6) suggests that astrocytes and microglia homeostasis critically depend on the presence of fully competent, functional chloride transporters.
Chemokine ligand 2 is a chemotactic factor that attracts monocytes, CD4+ T cells, natural killer cells, and dendritic cells to sites of inflammation (Carr et al., 1994). Increased CCL2 expression following inflammation or injury attracts leukocytes to mediate defense, cytokine release, and repair (Gschwandtner et al., 2019). Higher CCL2 expression in DRG neurons, as well as in the spinal cord, is associated with inflammatory pain and hypersensitivity (Menetski et al., 2007; Illias et al., 2018). We studied the effect of ClC-3-associated Cl–/H+ exchange on CCL2 expression levels in dorsal horn layers I–IV and found that CCL2 levels were increased in Clcn3–/–, Clcn4–/–, Clcn3E281Q/E281Q, and Clcn3E281Q/E281Q/Clcn4–/– mice (Figures 5B,Dc, 6B,F). A recent report demonstrated that CC12 is released via recycling endosomes and that changes in endo-lysosomal ion transport directly stimulate CCL2 release by promoting transfer through early and recycling endosomes (Plesch et al., 2018). ClC-3 isoforms are found in different organelles of the endo-lysosomal trafficking system, with ClC-3c present in recycling endosomes (Guzman et al., 2015; Comini et al., 2022). Taken together, our data suggest that impaired endosomal Cl–/H+ exchange causes neuroinflammation and pain in the Clcn3–/– mouse model via enhanced CCL2 release triggering an inflammatory reaction.
Many ion channels are sorted via the secretory pathway (Griffith, 2001; Swanwick et al., 2010; Capera et al., 2019) and recycled via clathrin-mediated endocytosis (Okuse et al., 2002; Liu et al., 2005; Swanwick et al., 2010; Conrad et al., 2018, 2021). ClC-3 has clathrin-binding motifs in its amino terminus (Zhao et al., 2007; Stauber and Jentsch, 2010) and, thus, might facilitate the endocytosis of membrane proteins and direct them to lysosomes (ClC-3b) or the recycling endosome (ClC-3c) (Guzman et al., 2015, 2017; Comini et al., 2022). In Clcn3E281Q/E281Q/Clcn4–/– mice, ClC-3-associated endosomal Cl–/H+ exchange does not occur. In contrast, Clcn3E281Q/E281Q animals have no obvious phenotype, likely because E281Q ClC-3 can still interact with and direct ClC-4 to the recycling endosome and lysosome (Guzman et al., 2017; Weinert et al., 2020). There are no phenotypic alterations in Clcn4–/–, but the lack of ClC-4 in the Clcn3E281Q/E281Q/Clcn4–/– mouse caused a strong CNS phenotype (Figure 7), with degenerated hippocampal CA1 and CA2 regions at the age, at which neurodegeneration is starting in the Clcn3–/– mouse. Retinal tissue appears to be more sensitive to reduced levels of Cl–/H+ transporters than the hippocampus. Whereas hippocampal regions are not affected in the Clcn3E281Q/E281Q, the reduced thickness of retinal neuronal tissue in these animals already evidences the tight dependency between the viability of the neurons and Cl–/H+ transporters in this CNS region. Recently, the Clcn3unc/unc knock-in mouse model was generated, carrying the E224A mutation in the “gating glutamate” that uncouples Cl– currents from H+ counter transport. Clcn3unc/unc mice do not have a severe phenotype, but in Clcn3unc/unc/Clcn4–/– animals hippocampal neurodegeneration can be seen in the third postnatal week (Weinert et al., 2020). Data from the Clcn3E281Q/E281Q/Clcn4–/– and Clcn3unc/unc/Clcn4–/– mouse models reinforce the importance of ClC-3 Cl–/H+ transport function for neuronal survival within the CNS in supraspinal brain regions, such as the hippocampus and retina.
Ablation or mutation of the genes encoding CLC-type Cl–/H+ exchangers has been linked to a variety of neurodevelopmental syndromes. Patients with these syndromes often have severe symptoms, thought to be caused by disturbed endosomal ion homeostasis. Here, we identified ClC-3-dependent cellular processes that are not coupled to its ion-exchange mechanism but are instead linked to a possible chaperone function. Our findings, summarized in Figure 8, identified ClC-3 as an integral part of the molecular machinery underlying age-related changes in neuronal sensitivity and excitability. We also demonstrated that altered Cl–/H+ transport may modify chemokine release and, thus, regulate inflammatory processes. Modulation of inflammatory responses by CLC-type transporter functions may be responsible for the severe phenotype of engineered animal models and may contribute to symptom severity in patients with CLC-linked neurological diseases.
Data availability statement
The original contributions presented in this study are included in the article/Supplementary material, further inquiries can be directed to the corresponding author.
Ethics statement
The animal study was reviewed and approved by German Animal Welfare Act (TierSchG § § 7–9) and State Agency for Nature, Environment and Consumer Protection, North Rhine Westphalia, and the local Animal Protection Committee, file numbers 84-02.04.2015.A108 and 84-02.04.2015.A307.
Author contributions
JS-M performed the experiments, analyzed the data, and drafted the manuscript. AW, CB, and MS performed the animal behavior test and analyzed the data. SB-P performed the Western blot experiments. JG and FM performed the retina and hippocampal immunostainings experiments. AL provided the macro for VGSC analyses. CF supervised the research and wrote the manuscript. RG conceived the idea, supervised the research, and wrote the manuscript. All authors contributed to the article and approved the submitted version.
Funding
This work was supported by the German Research Foundation (DFG) (GU 2042/2–1) to RG.
Acknowledgments
We thank Dr. T. Jentsch for providing the ClCn3–/– mouse model; Dr. Karlijn van Aerde and Dr. Dirk Feldmeyer for giving the Igor-based macro used to analyze AP data; Dr. Ute Becherer and Margaret Klose for providing the protocol used for extraction and culture of DRG neurons; and Verena Graf for assisting with the animal behavior experiments. We thank Dr. Juan Carlos Valdelamar-Villegas for helpful discussion. We are grateful to the staff of our animal facility and to Nicola Kornadt-Beck for her invaluable support in all aspects of animal work.
Conflict of interest
The authors declare that the research was conducted in the absence of any commercial or financial relationships that could be construed as a potential conflict of interest.
Publisher’s note
All claims expressed in this article are solely those of the authors and do not necessarily represent those of their affiliated organizations, or those of the publisher, the editors and the reviewers. Any product that may be evaluated in this article, or claim that may be made by its manufacturer, is not guaranteed or endorsed by the publisher.
Supplementary material
The Supplementary Material for this article can be found online at: https://www.frontiersin.org/articles/10.3389/fncel.2022.920075/full#supplementary-material
References
Abbadie, C., Taylor, B. K., Peterson, M. A., and Basbaum, A. I. (1997). Differential contribution of the two phases of the formalin test to the pattern of c-fos expression in the rat spinal cord: Studies with remifentanil and lidocaine. Pain 69, 101–110. doi: 10.1016/s0304-3959(96)03285x
Accardi, A., Walden, M., Nguitragool, W., Jayaram, H., Williams, C., and Miller, C. (2005). Separate ion pathways in a Cl-/H+ exchanger. J. Gen. Physiol. 126, 563–570. doi: 10.1085/jgp.200509417
Amaya, F., Izumi, Y., Matsuda, M., and Sasaki, M. (2013). Tissue injury and related mediators of pain exacerbation. Curr. Neuropharmacol. 11, 592–597. doi: 10.2174/1570159X11311060003
Bennett, D. L., Clark, A. J., Huang, J., Waxman, S. G., and Dib-Hajj, S. D. (2019). The role of voltage-gated sodium channels in pain signaling. Physiol. Rev. 99, 1079–1151. doi: 10.1152/physrev.00052.2017
Bernal, L. (2018). Insights into the contribution of voltage-gated sodium channel 1.7 to paclitaxel-induced neuropathy. J. Neurosci. 38, 6025–6027. doi: 10.1523/Jneurosci.0692-18.2018
Berta, T., Poirot, O., Pertin, M., Ji, R. R., Kellenberger, S., and Decosterd, I. (2008). Transcriptional and functional profiles of voltage-gated Na+ channels in injured and non-injured DRG neurons in the SNI model of neuropathic pain. Mol. Cell. Neurosci. 37, 196–208. doi: 10.1016/j.mcn.2007.09.007
Blair, N. T., and Bean, B. P. (2002). Roles of tetrodotoxin (TTX)-sensitive Na+ current, TTX-resistant Na+ current, and Ca2+ current in the action potentials of nociceptive sensory neurons. J. Neurosci. 22, 10277–10290. doi: 10.1523/JNEUROSCI.22-23-10277.2002
Bose, S., He, H., and Stauber, T. (2021). Neurodegeneration upon dysfunction of endosomal/lysosomal CLC chloride transporters. Front. Cell Dev. Biol. 9:639231. doi: 10.3389/fce11.2021.639231
Bost, A., Shaib, A. H., Schwarz, Y., Niemeyer, B. A., and Becherer, U. (2017). Large dense-core vesicle exocytosis from mouse dorsal root ganglion neurons is regulated by neuropeptide Y. J. Neurosci. 346, 1–13. doi: 10.1016/j.neuroscience.2017.01.006
Busserolles, J., Gasull, X., and Noël, J. (2020). “Potassium channels and pain,” in The Oxford Handbook of the Neurobiology of Pain, ed. P. J. N. Wood (Oxford University Press).
Capera, J., Serrano-Novillo, C., Navarro-Perez, M., Cassinelli, S., and Felipe, A. (2019). The potassium channel odyssey: Mechanisms of traffic and membrane arrangement. Int. J. Mol. Sci. 20, 734. doi: 10.3390/ijms20030734
Carr, M. W., Roth, S. J., Luther, E., Rose, S. S., and Springer, T. A. (1994). Monocyte chemoattractant protein 1 acts as a T-lymphocyte chemoattractant. Proc. Natl. Acad. Sci. U.S.A. 91, 3652–3656. doi: 10.1073/pnas.91.9.3652
Chen, L., Huang, J., Zhao, P., Persson, A. K., Dib-Hajj, F. B., Cheng, X., et al. (2018). Conditional knockout of NaV1.6 in adult mice ameliorates neuropathic pain. Sci. Rep. 8:3845. doi: 10.1038/s41598-018-22216-w.
Comini, M., Sierra-Marquez, J., Guzman, G., Franzen, A., Willuweit, A., Katona, I., et al. (2022). CLC anion/proton exchangers regulate secretory vesicle filling and granule exocytosis in chromaffin cells. J. Neurosci. 42, 3080–3095. doi: 10.1523/JNEUROSCI.2439-21.2022.
Conner, L. B., Alvarez, P., Bogen, O., and Levine, J. D. (2016). Role of Kv4.3 in vibration-induced muscle pain in the rat. J. Pain 17, 444–450. doi: 10.1016/j.jpain.2015.12.007
Conrad, R., Kortzak, D., Guzman, G. A., Miranda-Laferte, E., and Hidalgo, P. (2021). Ca(V) β controls the endocytic turnover of Ca(V) 1.2 L-type calcium channel. Traffic 22, 180–193. doi: 10.1111/tra.12788
Conrad, R., Stolting, G., Hendriks, J., Ruello, G., Kortzak, D., Jordan, N., et al. (2018). Rapid turnover of the cardiac L-type CaV1.2 channel by endocytic recycling regulates its cell surface availability. iScience 7, 1–15. doi: 10.1016/j.isci.2018.08.012
Cummins, T. R., and Waxman, S. G. (1997). Downregulation of tetrodotoxin-resistant sodium currents and upregulation of a rapidly repriming tetrodotoxin-sensitive sodium current in small spinal sensory neurons after nerve injury. J. Neurosci. 17, 3503–3514. doi: 10.1523/JNEUROSCI.17-10-03503.1997
Dib-Hajj, S. D., Cummins, T. R., Black, J. A., and Waxman, S. G. (2010). Sodium channels in normal and pathological pain. Annu. Rev. Neurosci. 33, 325–347. doi: 10.1146/annurev-neuro-060909-153234
Dickerson, L. W., Bonthius, D. J., Schutte, B. C., Yang, B., Barna, T. J., Bailey, M. C., et al. (2002). Altered GABAergic function accompanies hippocampal degeneration in mice lacking ClC-3 voltage-gated chloride channels. Brain Res. 958, 227–250. doi: 10.1016/s0006-8993(02)03519-9
Duan, K.-Z., Xu, Q., Zhang, X.-M., Zhao, Z.-Q., Mei, Y.-A., and Zhang, Y.-Q. (2012). Targeting A-type K+ channels in primary sensory neurons for bone cancer pain in a rat model. Pain 153, 562–574. doi: 10.1016/j.pain.2011.11.020
Duncan, A. R., Polovitskaya, M. M., Gaitan-Penas, H., Bertelli, S., VanNoy, G. E., Grant, P. E., et al. (2021). Unique variants in CLCN3, encoding an endosomal anion/proton exchanger, underlie a spectrum of neurodevelopmental disorders. Am. J. Hum. Genet. 108, 1450–1465. doi: 10.1016/j.ajhg.2021.06.003
Fischer, B. D., Ho, C., Kuzin, I., Bottaro, A., and O’Leary, M. E. (2017). Chronic exposure to tumor necrosis factor in vivo induces hyperalgesia, upregulates sodium channel gene expression and alters the cellular electrophysiology of dorsal root ganglion neurons. Neurosci. Lett. 653, 195–201. doi: 10.1016/j.neulet.2017.05.004
Griffith, L. C. (2001). Potassium channels: The importance of transport signals. Curr. Biol. 11, R226–R228. doi: 10.1016/S0960-9822(01)00111-7
Gschwandtner, M., Derler, R., and Midwood, K. S. (2019). More than just attractive: How CCL2 influences myeloid cell behavior beyond chemotaxis. Front. Immunol. 10:2759. doi: 10.3389/fimmu.2019.02759
Guzman, R. E., Bungert-Plümke, S., Franzen, A., and Fahlke, C. (2017). Preferential association with ClC-3 permits sorting of ClC-4 into endosomal compartments. J. Biol. Chem. 292, 19055–19065. doi: 10.1074/jbc.M117.801951
Guzman, R. E., Grieschat, M., Fahlke, C., and Alekov, A. K. (2013). ClC-3 is an intracellular chloride/proton exchanger with large voltage-dependent nonlinear capacitance. ACS Chem. Neurosci. 4, 994–1003. doi: 10.1021/cn400032z
Guzman, R. E., Miranda-Laferte, E., Franzen, A., and Fahlke, C. (2015). Neuronal ClC-3 splice variants differ in subcellular localizations, but mediate identical transport functions. J. Biol. Chem. 290, 25851–25862. doi: 10.1074/jbc.M115.668186
Guzman, R. E., Sierra-Marquez, J., Bungert-Plumke, S., Franzen, A., and Fahlke, C. (2022). Functional characterization of CLCN4 variants associated with X-linked intellectual disability and epilepsy. Front. Mol. Neurosci. 15:872407. doi: 10.3389/fnmol.2022.872407
He, H., Cao, X., Yin, F., Wu, T., Stauber, T., and Peng, J. (2021a). West syndrome caused by a chloride/proton exchange-uncoupling CLCN6 mutation related to autophagic-lysosomal dysfunction. Mol. Neurobiol. 58, 2990–2999. doi: 10.1007/s12035-021-02291-3
He, H., Guzman, R. E., Cao, D., Sierra-Marquez, J., Yin, F., Fahlke, C., et al. (2021b). The molecular and phenotypic spectrum of CLCN4-related epilepsy. Epilepsia 6, 1401–1415. doi: 10.1111/epi.16906
Hoerauf, W. W., Cazares, V. A., Subramani, A., and Stuenkel, E. L. (2015). Efficient transfection of dissociated mouse chromaffin cells using small-volume electroporation. Cytotechnology 67, 573–583. doi: 10.1007/s10616-014-9699-y
Hu, H., Haas, S. A., Chelly, J., Van Esch, H., Raynaud, M., de Brouwer, A. P., et al. (2016). X-exome sequencing of 405 unresolved families identifies seven novel intellectual disability genes. Mol. Psychiat. 21, 133–148. doi: 10.1038/mp.2014.193
Hunskaar, S., and Hole, K. (1987). The formalin test in mice: dissociation between inflammatory and non-inflammatory pain. Pain 30, 103–114. doi: 10.1016/0304-3959(87)90088-1
Illias, A. M., Gist, A. C., Zhang, H., Kosturakis, A. K., and Dougherty, P. M. (2018). Chemokine CCL2 and its receptor CCR2 in the dorsal root ganglion contribute to oxaliplatin-induced mechanical hypersensitivity. Pain 159, 1308–1316. doi: 10.1097/j.pain.0000000000001212
Jentsch, T. J., and Pusch, M. (2018). CLC chloride channels and transporters: Structure, function, physiology, and disease. Physiol. Rev. 98, 1493–1590. doi: 10.1152/physrev.00047.2017
Kasper, D., Planells-Cases, R., Fuhrmann, J. C., Scheel, O., Zeitz, O., Ruether, K., et al. (2005). Loss of the chloride channel ClC-7 leads to lysosomal storage disease and neurodegeneration. EMBO J. 24, 1079–1091. doi: 10.1038/sj.emboj.7600576
Kornak, U., Kasper, D., Bosl, M. R., Kaiser, E., Schweizer, M., Schulz, A., et al. (2001). Loss of the ClC-7 chloride channel leads to osteopetrosis in mice and man. Cell 104, 205–215. doi: 10.1016/s0092-8674(01)00206-9
Lampert, A., O’Reilly, A. O., Reeh, P., and Leffler, A. (2010). Sodium channelopathies and pain. Pflugers Arch. 460, 249–263. doi: 10.1007/s00424-009-0779-3
Lamprecht, M. R., Sabatini, D. M., and Carpenter, A. E. (2007). CellProfiler: Free, versatile software for automated biological image analysis. Biotechniques 42, 71–75. doi: 10.2144/000112257
Lan, X., Han, X., Li, Q., Yang, Q.-W., and Wang, J. (2017). Modulators of microglial activation and polarization after intracerebral haemorrhage. Nat. Rev. Neurol. 13, 420–433. doi: 10.1038/nrneur01.2017.69
Laumet, G., Garriga, J., Chen, S.-R., Zhang, Y., Li, D.-P., Smith, T. M., et al. (2015). G9a is essential for epigenetic silencing of K+ channel genes in acute-to-chronic pain transition. Nat. Neurosci. 18, 1746–1755. doi: 10.1038/nn.4165
Lazarus, M. S., and Huang, Z. J. (2011). Distinct maturation profiles of perisomatic and dendritic targeting GABAergic interneurons in the mouse primary visual cortex during the critical period of ocular dominance plasticity. J. Neurophysiol. 106, 775–787. doi: 10.1152/jn.00729.2010
Lein, E. S., Hawrylycz, M. J., Ao, N., Ayres, M., Bensinger, A., Bernard, A., et al. (2007). Genome-wide atlas of gene expression in the adult mouse brain. Nature 445, 168–176. doi: 10.1038/nature05453
Liu, C., Cummins, T. R., Tyrrell, L., Black, J. A., Waxman, S. G., and Dib-Hajj, S. D. (2005). CAP-1A is a novel linker that binds clathrin and the voltage-gated sodium channel Na(v)1.8. Mol. Cell. Neurosci. 28, 636–649. doi: 10.1016/j.mcn.2004.11.007
Liu, Y., Schirra, C., Edelmann, L., Matti, U., Rhee, J., Hof, D., et al. (2010). Two distinct secretory vesicle-priming steps in adrenal chromaffin cells. J. Cell Biol. 190:1067. doi: 10.1083/jcb.201001164
Marics, I., Malapert, P., Reynders, A., Gaillard, S., and Moqrich, A. (2014). Acute heat-evoked temperature sensation is impaired but not abolished in mice lacking TRPV1 and TRPV3 channels. PLoS One 9:e99828. doi: 10.1371/journal.pone.0099828
McQuin, C., Goodman, A., Chernyshev, V., Kamentsky, L., Cimini, B. A., Karhohs, K. W., et al. (2018). CellProfiler 3.0: Next-generation image processing for biology. PLoS Biol. 16:e2005970. doi: 10.1371/journal.pbi0.2005970
Meents, J. E., and Lampert, A. (2016). “Studying sodium channel gating in heterologous expression systems,” in Advanced Patch-Clamp Analysis for Neuroscientists, ed. A. Korngreen (New York, NY: Springer), 37–65.
Menetski, J., Mistry, S., Lu, M., Mudgett, J. S., Ransohoff, R. M., DeMartino, J. A., et al. (2007). Mice overexpressing chemokine ligand 2 (CCL2) in astrocytes display enhanced nociceptive responses. J. Neurosci. 149, 706–714. doi: 10.1016/j.neuroscience.2007.08.014
Mika, J., Zychowska, M., Popiolek-Barczyk, K., Rojewska, E., and Przewlocka, B. (2013). Importance of glial activation in neuropathic pain. Eur. J. Pharmacol. 716, 106–119. doi: 10.1016/j.ejphar.2013.01.072
Okuse, K., Malik-Hall, M., Baker, M. D., Poon, W. Y., Kong, H., Chao, M. V., et al. (2002). Annexin II light chain regulates sensory neuron-specific sodium channel expression. Nature 417, 653–656. doi: 10.1038/nature00781
Palmer, E. E., Stuhlmann, T., Weinert, S., Haan, E., Van Esch, H., Holvoet, M., et al. (2018). De novo and inherited mutations in the X-linked gene CLCN4 are associated with syndromic intellectual disability and behavior and seizure disorders in males and females. Mol. Psychiat. 23, 222–230. doi: 10.1038/mp.2016.135
Pang, R.-P., Xie, M.-X., Yang, J., Shen, K.-F., Chen, X., Su, Y.-X., et al. (2016). Downregulation of ClC-3 in dorsal root ganglia neurons contributes to mechanical hypersensitivity following peripheral nerve injury. Neuropharmacology 110, 181–189. doi: 10.1016/j.neuropharm.2016.07.023
Petersson, M. E., Obreja, O., Lampert, A., Carr, R. W., Schmelz, M., and Fransen, E. (2014). Differential axonal conduction patterns of mechano-sensitive and mechano-insensitive nociceptors–a combined experimental and modelling study. PLoS One 9:e103556. doi: 10.1371/journal.pone.0103556
Plesch, E., Chen, C. C., Butz, E., Scotto Rosato, A., Krogsaeter, E. K., Yinan, H., et al. (2018). Selective agonist of TRPML2 reveals direct role in chemokine release from innate immune cells. Elife 7:39720. doi: 10.7554/eLife.39720
Poet, M., Kornak, U., Schweizer, M., Zdebik, A. A., Scheel, O., Hoelter, S., et al. (2006). Lysosomal storage disease upon disruption of the neuronal chloride transport protein ClC-6. Proc. Natl. Acad. Sci. U.S.A. 103, 13854–13859. doi: 10.1073/pnas.0606137103
Priestley, J. V. (2009). “Neuropeptides: Sensory systems,” in Encyclopedia of Neuroscience, ed. L. R. Squire (Oxford: Academic Press), 935–943.
Rasband, M. N., Park, E. W., Vanderah, T. W., Lai, J., Porreca, F., and Trimmer, J. S. (2001). Distinct potassium channels on pain-sensing neurons. Proc. Natl. Acad. Sci. U.S.A. 98, 13373–13378. doi: 10.1073/pnas.231376298
Ren, K., and Dubner, R. (2008). Neuron-glia crosstalk gets serious: Role in pain hypersensitivity. Curr. Opin. Anaest. 21, 570–579. doi: 10.1097/AC0.0b013e32830edbdf
Rohrbough, J., Nguyen, H. N., and Lamb, F. S. (2018). Modulation of ClC-3 gating and proton/anion exchange by internal and external protons and the anion selectivity filter. J. Physiol. 596, 4091–4119. doi: 10.1113/jp276332
Rueden, C. T., Schindelin, J., Hiner, M. C., DeZonia, B. E., Walter, A. E., Arena, E. T., et al. (2017). ImageJ2: ImageJ for the next generation of scientific image data. BMC Bioinform. 18:529. doi: 10.1186/s12859-017-1934-z
Rush, A. M., Cummins, T. R., and Waxman, S. G. (2007). Multiple sodium channels and their roles in electrogenesis within dorsal root ganglion neurons. J. Physiol. 579, 1–14. doi: 10.1113/jphysi01.2006.121483
Schindelin, J., Arganda-Carreras, I., Frise, E., Kaynig, V., Longair, M., Pietzsch, T., et al. (2012). Fiji: An open-source platform for biological-image analysis. Nat. Meth. 9, 676–682. doi: 10.1038/nmeth.2019
Scott, B., Leu, J., and Cinader, B. (1988). Effects of aging on neuronal electrical membrane properties. Mech. Ageing Dev. 44, 203–214. doi: 10.1016/0047-6374(88)90022-x
Seitz, V., Stötzner, P., Labuz, D., and Machelska, H. (2021). “Patch clamp analysis of opioid-induced Kir3 currents in mouse peripheral sensory neurons following nerve injury,” in Opioid Receptors: Methods and Protocols, ed. S. M. Spampinato (New York, NY: Springer), 127–137. doi: 10.1007/978-1-0716-0884-5_12
Stauber, T., and Jentsch, T. J. (2010). Sorting motifs of the endosomal/lysosomal CLC chloride transporters*. J. Biol. Chem. 285, 34537–34548. doi: 10.1074/jbc.M110.162545
Stobrawa, S. M., Breiderhoff, T., Takamori, S., Engel, D., Schweizer, M., Zdebik, A. A., et al. (2001). Disruption of ClC-3, a chloride channel expressed on synaptic vesicles, leads to a loss of the hippocampus. Neuron 29, 185–196. doi: 10.1016/S0896-6273(01)00189-1
Stucky, C. L. (2007). “IB4-positive neurons, role in inflammatory pain,” in Encyclopedia of Pain, eds R. F. Schmidt and W. D. Willis (Berlin, Heidelberg: Springer), 952–955.
Suaudeau, C., do-Rego, J.-C., and Costentin, J. (2005). Modifications in avoidance reactions of mice, on a second exposure to the hot plate, resist to various amnesia-inducing treatments. Cogn. Brain Res. 25, 339–347. doi: 10.1016/j.cogbrainres.2005.06.007
Swanwick, R. S., Pristerá, A., and Okuse, K. (2010). The trafficking of Na(V)1.8. Neurosci. Lett. 486, 78–83. doi: 10.1016/j.neulet.2010.08.074
Taylor, B. K., Peterson, M. A., and Basbaum, A. I. (1995). Persistent cardiovascular and behavioral nociceptive responses to subcutaneous formalin require peripheral nerve input. J. Neurosci. 15, 7575–7584. doi: 10.1523/JNEUROSCI.15-11-07575.1995
Tigerholm, J., Petersson, M. E., Obreja, O., Eberhardt, E., Namer, B., Weidner, C., et al. (2015). C-fiber recovery cycle supernormality depends on ion concentration and ion channel permeability. Biophys. J. 108, 1057–1071. doi: 10.1016/j.bpj.2014.12.034
Tjølsen, A., Berge, O.-G., Hunskaar, S., Rosland, J. H., and Hole, K. (1992). The formalin test: An evaluation of the method. Pain 51, 5–17. doi: 10.1016/0304-3959(92)90003-T
Vallejo, R., Tilley, D. M., Vogel, L., and Benyamin, R. (2010). The role of glia and the immune system in the development and maintenance of neuropathic pain. Pain Pract. 10, 167–184. doi: 10.1111/j.1533-2500.2010.00367.x
Veeramah, K. R., Johnstone, L., Karafet, T. M., Wolf, D., Sprissler, R., Salogiannis, J., et al. (2013). Exome sequencing reveals new causal mutations in children with epileptic encephalopathies. Epilepsia 54, 1270–1281. doi: 10.1111/epi.12201
Vydyanathan, A., Wu, Z. Z., Chen, S. R., and Pan, H. L. (2005). A-type voltage-gated K+ currents influence firing properties of isolectin B4-positive but not isolectin B4-negative primary sensory neurons. J. Neurophysiol. 93, 3401–3409. doi: 10.1152/jn.01267.2004
Wang, S., and Albers, K. M. (2009). Behavioral and cellular level changes in the aging somatosensory system. Ann. N.Y Acad. Sci. 1170, 745–749. doi: 10.1111/j.1749-6632.2009.04011.x
Weinert, S., Gimber, N., Deuschel, D., Stuhlmann, T., Puchkov, D., Farsi, Z., et al. (2020). Uncoupling endosomal CLC chloride/proton exchange causes severe neurodegeneration. EMBO J. 39:e103358. doi: 10.15252/embj.2019103358
Yoshikawa, M., Uchida, S., Ezaki, J., Rai, T., Hayama, A., Kobayashi, K., et al. (2002). CLC-3 deficiency leads to phenotypes similar to human neuronal ceroid lipofuscinosis. Genes Cells 7, 597–605. doi: 10.1046/j.1365-2443.2002.00539
Keywords: ClC-3, chloride-proton exchanger, neuronal excitability, pain, microglia activation, action potential, DRG
Citation: Sierra-Marquez J, Willuweit A, Schöneck M, Bungert-Plümke S, Gehlen J, Balduin C, Müller F, Lampert A, Fahlke C and Guzman RE (2022) ClC-3 regulates the excitability of nociceptive neurons and is involved in inflammatory processes within the spinal sensory pathway. Front. Cell. Neurosci. 16:920075. doi: 10.3389/fncel.2022.920075
Received: 20 April 2022; Accepted: 28 July 2022;
Published: 24 August 2022.
Edited by:
Luigi Catacuzzeno, University of Perugia, ItalyReviewed by:
Ilenio Servettini, Università di Napoli Federico II, ItalyAntonio Michelucci, University of Perugia, Italy
Copyright © 2022 Sierra-Marquez, Willuweit, Schöneck, Bungert-Plümke, Gehlen, Balduin, Müller, Lampert, Fahlke and Guzman. This is an open-access article distributed under the terms of the Creative Commons Attribution License (CC BY). The use, distribution or reproduction in other forums is permitted, provided the original author(s) and the copyright owner(s) are credited and that the original publication in this journal is cited, in accordance with accepted academic practice. No use, distribution or reproduction is permitted which does not comply with these terms.
*Correspondence: Raul E. Guzman, r.guzman@fz-juelich.de