Lagomorpha as a Model Morphological System
- 1Department of Anatomy, Western University of Health Sciences, Pomona, CA, United States
- 2Laboratory of Biotechnologies Related to Animal Reproduction, Institute of Veterinary Sciences, Blida 1 University, Blida, Algeria
- 3Key Laboratory of Vertebrate Evolution and Human Origins, Institute of Vertebrate Paleontology and Paleoanthropology, Chinese Academy of Sciences, Beijing, China
- 4CAS Center for Excellence in Life and Paleoenvironment, Beijing, China
- 5Institute of Paleobiology, Polish Academy of Sciences, Warsaw, Poland
- 6Key Laboratory of Zoological Systematics and Evolution, Institute of Zoology, Chinese Academy of Sciences, Beijing, China
- 7Borissiak Paleontological Institute, Russian Academy of Sciences, Moscow, Russia
- 8Department of Anthropology, University of Toronto Scarborough, Toronto, ON, Canada
- 9Division of Paleontology, American Museum of Natural History, New York, NY, United States
- 10New York Consortium in Evolutionary Primatology, New York, NY, United States
- 11Department of Biology, Faculty of Science, Gorgan, Golestan, Iran
- 12Department of Biological Sciences, Vanderbilt University, Nashville, TN, United States
- 13Abteilung Messelforschung und Mammalogie, Senckenberg Forschungsinstitut und Naturmuseum Frankfurt, Frankfurt am Main, Germany
- 14Departments of Biological Sciences, Aerospace and Mechanical Engineering, and Anthropology, University of Notre Dame, Notre Dame, IN, United States
- 15Evolutionary Morphology and Biomechanics Group, Institute of Life Course and Medical Sciences, University of Liverpool, Liverpool, United Kingdom
- 16School of Biological Sciences, The University of Adelaide, Adelaide, SA, Australia
- 17Roy M. Huffington Department of Earth Sciences, Southern Methodist University, Dallas, TX, United Stats
- 18Department of Cell Biology, University of Texas Southwestern Medical Center, Dallas, TX, United States
Due to their global distribution, invasive history, and unique characteristics, European rabbits are recognizable almost anywhere on our planet. Although they are members of a much larger group of living and extinct mammals [Mammalia, Lagomorpha (rabbits, hares, and pikas)], the group is often characterized by several well-known genera (e.g., Oryctolagus, Sylvilagus, Lepus, and Ochotona). This representation does not capture the extraordinary diversity of behavior and form found throughout the order. Model organisms are commonly used as exemplars for biological research, but there are a limited number of model clades or lineages that have been used to study evolutionary morphology in a more explicitly comparative way. We present this review paper to show that lagomorphs are a strong system in which to study macro- and micro-scale patterns of morphological change within a clade that offers underappreciated levels of diversity. To this end, we offer a summary of the status of relevant aspects of lagomorph biology.
Introduction
Lagomorpha (rabbits, hares, and pikas) is a globally distributed (barring Antarctica) extant mammalian order within the larger superorder Euarchontoglires (rodents, lagomorphs, treeshrews, colugos, and primates) (Murphy et al., 2001). The order consists of herbivorous species across two extant families, the Ochotonidae (pikas) and the Leporidae (rabbits and hares) (Stock, 1976;
Forsyth et al., 2005; Hoffmann and Smith, 2005; Burgin et al., 2018; Smith et al., 2018; Figure 1). There are presently 12 living lagomorph genera recognized, subsuming 108 recognized species (see Burgin et al., 2018 for a recent treatment). These genera are distributed unequally between two families; the Leporidae contains 11 genera, the most well-known being Lepus (hares and jackrabbits), Sylvilagus (cottontails), and Oryctolagus (European rabbit) (Naff and Craig, 2012; Graham, 2015) while Ochotonidae includes only a single living genus, Ochotona (pikas) (Hoffmann and Smith, 2005; Ge et al., 2012; Smith et al., 2018; Figure 2).
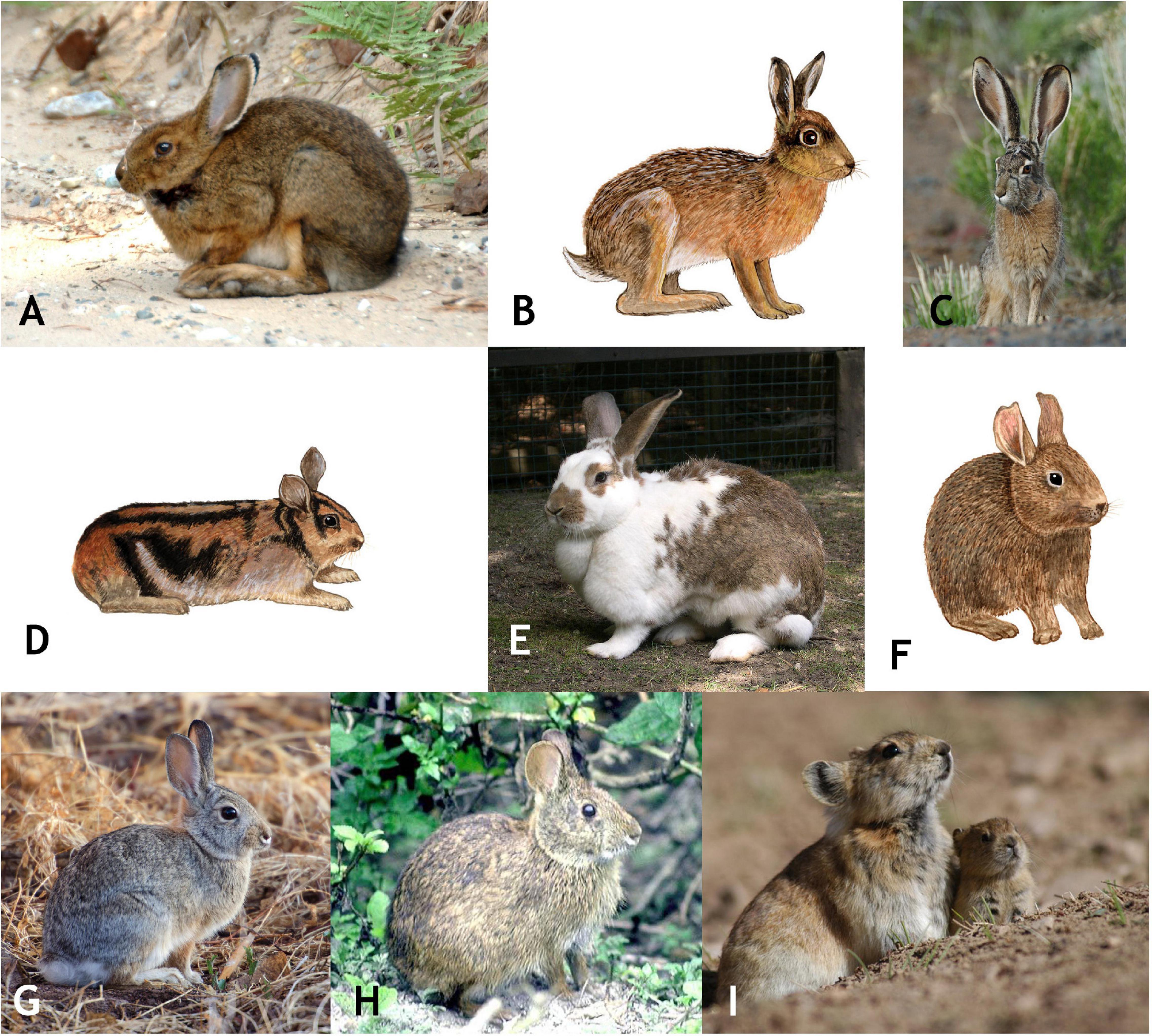
Figure 1. A representative selection of extant lagomorphs, including: (A) Lepus americanus (snowshoe hare); (B) Lepus europaeus (European hare); (C) Lepus californicus (Black-tailed jackrabbit); (D) Nesolagus timminsi (Annamite striped rabbit); (E) Oryctolagus cuniculus (European rabbit); (F) Romerolagus diazi (Volcano rabbit); (G) Sylvilagus audubonii (Audobon’s cottontail); (H) Sylvilagus palustris (Marsh rabbit); (I) Ochotona curzoniae (Black-lipped pika). All images from Myers et al. (2020).
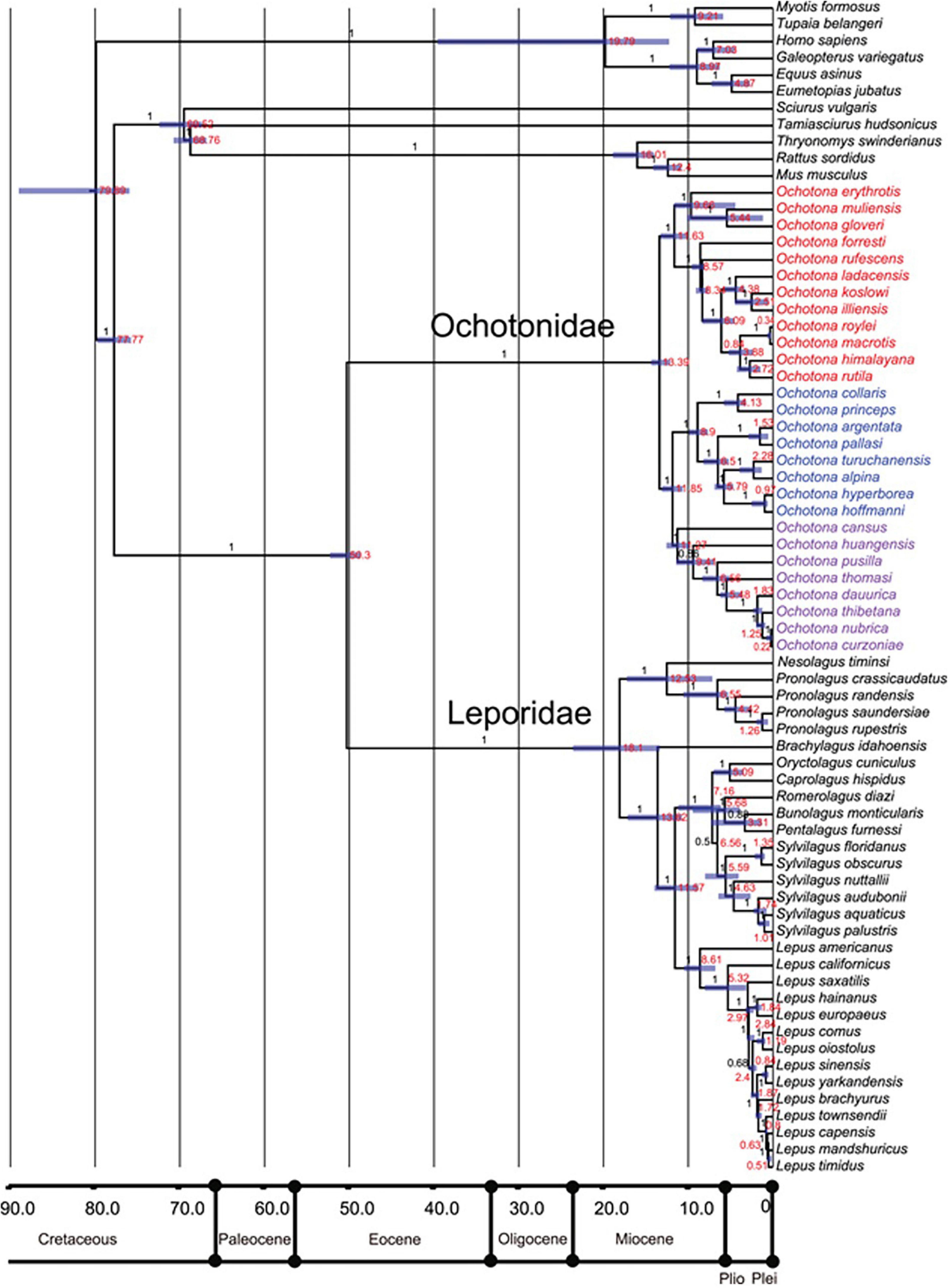
Figure 2. Phylogeny of selected extant lagomorph species by Ge et al. (2013).
Lagomorphs have featured prominently in the set of non-human model organisms that have driven many advances within the biological sciences over the last century, particularly to understand genetic, genomic, or developmental processes that drive biological change (Leonelli and Ankeny, 2013). Recent research on understanding the genome level architecture of life has included the European rabbit (Oryctolagus cuniculus), the mountain hare (Lepus timidus), the snowshoe hare (Lepus americanus), and the American pika (Ochotona princeps) (Marques et al., 2020). Oryctolagus and Ochotona have often served as the model lagomorphs in larger scale comparative studies of mammals (e.g., Sánchez-Villagra et al., 2017; Hecker et al., 2019). Though such studies have revealed much about broad patterns among mammals, often in an evolutionary context, there has been little comprehensive comparative work done within the lagomorph clade.
Monaghan (2014, p. 1019), through the lens of behavioral ecology, argue that the modern concept of model organisms has limited some insights within the biological sciences away from a more intentional focus “to understand the processes responsible for the diversity that we see in animal form and function.” Because such mechanisms often can be best understood by studying lineages and clades, we argue that Lagomorpha represent an ideal clade with which to explore these processes. Chapman and Flux (1990) have made such an argument for lagomorphs, which we expand here to include current scientific standings.
Among vertebrates, the radiations of anole lizards (Anolis) are a strong example of a clade that has served as a model system to study integrative processes in evolution and adaptive radiations (Sanger and Kircher, 2017). Extensive work on Anolis has included the historical, genetic, and developmental basis of anole diversity (e.g., Losos, 2011; Sanger et al., 2012; Sherratt et al., 2015; Corbett-Detig et al., 2020; Velasco et al., 2020). Though Anolis represents a strong comparative system, it is limited at scales that span longer geological periods. Lagomorpha are anchored by an extensively used model organism, the European rabbit (O. cuniculus), but include a rich fossil record that goes back to the Paleocene, a diverse group of living lineages, significant morphological and ecological disparity, and functional variation in multiple key aspects of behavioral biology. The goal of this review is to summarize key biological features of the lagomorph clade to highlight how they represent an ideal group to investigate both macro- and micro-level questions in morphological biology.
Lagomorph Biology
Rabbits and hares are found in forests, in open scrub, or savannah in Eurasia, Africa, and North, Central, and northern South America. Additionally, the European rabbit and hare have been introduced into Australia and South America (Chapman and Flux, 1990; Ge et al., 2013). Lagomorphs are hind-gut fermenters and require cecotrophy as well as at least a 15% crude fiber diet to maintain gastrointestinal health. Leporids are typically crepuscular and most active during the twilight hours at sunrise and sunset. They eat a wide variety of herbaceous material and grasses represent 30% of the plant food species ingested (Ge et al., 2013; Delaney et al., 2018). Pikas require a more specialized environment than hares and rabbits. They are most often found at high elevations in cold semiarid regions (Delaney et al., 2018). Pikas are distributed mainly throughout Asia, Eastern Europe, and western North America (Berkovitz and Shellis, 2018). They consume a wide variety of herbaceous plants, but grasses are a much smaller component of the diet than in that of rabbits and hares (Ge et al., 2013). The eyes of lagomorphs are laterally positioned, providing a circular field of vision (Delaney et al., 2018).
Among lagomorphs, the pikas are generally smaller (12–25 cm long; 100–400 g), with short limbs and small ears compared to their larger rabbit and hare counterparts (Ge et al., 2013; Delaney et al., 2018). Rabbits and hares have a short tail and lack or have minimal sexual dimorphism (e.g., Chapman and Ceballos, 1990; Fa and Bell, 1990; though see Orr, 1940 on sexual dimorphism in Sylvilagus); pikas lack an external tail and both sexes have a cloaca-like structure and lack sexual dimorphism (Graham, 2015). Most leporid males have testes located in a scrotum in front of the penis and females possess two to five pairs of mammary glands. Embryonically, the mammary glands develop from the mammary line (ridge), as is typical for placentals. However, it was found that, in the European rabbit, the anterior pair of mammary glands appear separately above the mammary ridge, in the axilla region of the forelimb (Propper, 1976). Gestation is 21–30 days in Ochotonidae and 24–55 days in Leporidae. Ochotonidae are typically altricial, though in some species the neonates are covered with fur. Leporidae show both patterns, altricial (Brachylagus idahoensis, Bunolagus monticularis, O. cuniculus, and Sylvilagus spp.) and precocial (Lepus spp.) species (Lissovsky, 2016). Pentalagus furnessi, Poelagus marjorita, Pronolagus rupestris, and Romerolagus diazi are altricial as well but the young are variable with respect to fur at birth. For some rare species of Pronolagus, Nesolagus spp., and Caprolagus hispidus, almost no information is yet available due to their rarity (Lissovsky, 2016; Schai-Braun and Hackländer, 2016). This is representative of our anatomical knowledge of lagomorphs, while there are comprehensive treaties on Oryctolagus cuniculus (Krause, 1884; Bensley, 1921; Barone et al., 1973) other species are much less studied.
Systematics
Within the 11 extant leporid genera there are approximately 75 species and 35 species in the single extant ochotonid genus, Ochotona (Hoffmann and Smith, 2005; Ge et al., 2012, 2013; Burgin et al., 2018). A summary of the fossil record of the Lagomorpha by Ge et al. (2013) includes about 45 genera and more than 190 species of Leporidae, and about 32 genera and 180 species of Ochotonidae (for both families, these are formally nominated taxa). The following sections highlight key features of these groups and the status of systematic relationships.
Key Craniodental Characters
Lagomorpha have been defined by a broad set of specific characters, most of which are related to mastication and locomotion, due somewhat to the relevant abundance of related osteological regions in the fossil record (e.g., López Martínez, 1985; Asher et al., 2005, 2019; Wible, 2007; Lopez-Martinez, 2008; Koenigswald et al., 2010). The living lagomorph families can be easily distinguished by several morphological characters. Besides obvious external anatomical traits such as size and shape of the outer ear and limb proportions, there exist discrete craniodental differences between the two families: e.g., proportions of the rostrum and absence or presence of fenestration and pitting of the os maxillare and bones of the posterior skull (Wood, 1940; Angermann et al., 1990; Wible, 2007). As in rodents, all lagomorphs have hypselodont (evergrowing) incisors, which are unreplaced deciduous teeth. They differ from rodents in having a second set of small permanent upper incisors. The dental formula in leporids is: I 2/1, C 0/0, P 3/2, M 3/3 with 28 total teeth and in ochotonids is: I 2/1, C 0/0, P 3/2, M 2/3 with 26 teeth (Graham, 2015; Delaney et al., 2018). See Wible (2007), Fostowicz-Frelik and Meng (2013), and Ruf (2014) for a review of lagomorph cranial literature. Soft tissue structures such as the cephalic arterial system (Bugge, 1974) also can be used for investigation of fossil species because adjacent bony structures (e.g., foramina, sulci) serve as proxies.
In contrast, taxonomy and systematic relationships at the genus and species level, and the phylogenetic position of certain fossil taxa, remain unresolved due to possible homoplastic evolution of certain characters (Robinson and Matthee, 2005; Kraatz et al., 2010; Fostowicz-Frelik and Meng, 2013; Asher et al., 2019). For instance, the premolar foramen was regarded as a synapomorphic character of Ochotonidae although a puzzling pattern among extant Lagomorpha became evident (Corbet, 1983: lateral palatal foramen); however, a study using broad taxon sampling including fossil species clearly revealed its diversity and homoplastic nature among Lagomorpha (Fostowicz-Frelik and Meng, 2013). In his comprehensive comparative description of the external craniomandibular anatomy of O. princeps, R. diazi and further selected Leporidae, Wible (2007) complemented and refined a character matrix comprising 229 traits including 92 craniomandibular characters (Meng et al., 2003; Asher et al., 2005). To date, intracranial structures are underrepresented in phylogenetic studies of Lagomorpha. Recent studies on the nasal and ear region defined new intracranial characters that can significantly contribute to a deeper understanding of lagomorph systematics, evolution, and morphofunction. For example, extant Ochotonidae and Leporidae differ significantly in the number of turbinals (Ruf, 2014). The former have a reduced number of olfactory turbinals and lack the interturbinal in the frontoturbinal recess. The turbinal pattern in the ethmoturbinal recess of Leporidae shows certain apomorphic character states (number of ethmo- and interturbinals) in several clades that can also be used for systematic purposes at the genus and species level (Ruf, 2014).
The middle ear morphology of extant Lagomorpha reveals unique family-specific patterns of the anterior attachment of the malleus by means of the processus anterior and its processus internus praearticularis; however, the phylogenetic polarization of this character still was pending (Maier et al., 2018). A first attempt to polarize the observed patterns could be achieved by the first high-resolution computed tomography (μCT) study on intracranial structures in a fossil lagomorph; Palaeolagus haydeni reveals that early ontogenetic stages of Ochotona may represent the plesiomorphic lagomorph pattern (Ruf et al., 2021). This clearly shows the potential of μCT investigations of fossil Leporidae and Ochotonidae for elucidating the evolution of intracranial characters.
Traditionally, dental characters play a major role in lagomorph taxonomy and systematics, especially in fossil species, and there is extensive literature summarizing this important system (e.g., Dawson, 1958; Hibbard, 1963; White, 1991; Averianov and Tesakov, 1997; Patnaik, 2002; Kraatz et al., 2010; Winkler and Tomida, 2011). The occlusal pattern of anterior premolars (particularly p3) is highly diagnostic, even on a lower taxonomic level. However, although most individuals within an extant or fossil population will have the diagnostic pattern, there often are individuals (in particular, younger ones with less occlusal wear) preserving a pattern that would suggest assignment to a different taxon (Hibbard, 1963). The masticatory pattern, also reflected in the occlusal morphology and the number of shearing blades of the cheek teeth, separates most Ochotonidae from all but two genera (Romerolagus diazi and Pronolagus) of extant Leporidae (Koenigswald et al., 2010). Beside systematic relationships and some species-specific patterns, this character complex provides insight into the evolution of functional adaptations. The lagicone structure, a complex enamel structure on the buccal occlusal surface of stem lagomorphs and certain Ochotonidae (López Martínez, 1985), becomes reduced in fossil European ochotonids; thus, the shearing function in pikas is increased. In most Leporidae the grinding function is enhanced by crenulation of specific enamel bands (Koenigswald et al., 2010). The two living lagomorph families also can be distinguished by the enamel pattern (schmelzmuster) of their incisors, a key character complex in terms of evolution and morphofunction of Glires (Martin, 1999, 2004). Generally, the incisors of Leporidae show a single-layered schmelzmuster in concert with multi-layered Hunter-Schreger bands (HSB). This pattern is derived from a double-layered schmelzmuster as observed in an undetermined leporid from the early Eocene of Kyrgyzstan and in some Mimotonidae. In contrast, Ochotonidae have a multi-layered schmelzmuster with modified HSB and enamel patterns differing in upper and lower incisors (Martin, 1999, 2004).
Key Postcranial Characters
Overall, the body-plan of lagomorphs is relatively uniform throughout their evolutionary history. There are two basic archetypes, which are represented by two extant families: the longer legged rabbits and hares, and shorter legged, rather stocky ochotonids. The ochotonid-like morphotype (or small, relatively short-limbed rabbits) dominated during the Paleogene, which suggests that true cursorial abilities appeared within lagomorphs later in the early Neogene. However, the structure of the lagomorph hindlimb with the closely connected tibia and fibula (fused already in the late Eocene Palaeolagus), and a unique direct calcaneo-fibular contact known from the Middle Paleocene duplicidentate of China (Fostowicz-Frelik, 2017) indicate cursorial adaptations since the groups’ inception. The calcaneus is known in several Eocene lagomorphs, including the Asian Dawsonolagus and Strenulagus (Li et al., 2007; Fostowicz-Frelik et al., 2015a) and North American Palaeolagus (Wood, 1940). In most Paleogene lagomorphs, calcaneal structure is similar to Ochotona; the latter is somewhat stockier (compared to that of leporids) with a proportionally shorter tuber and calcaneal body. The calcaneal canal is a striking synapomorphy of Lagomorpha (Bleefeld and Bock, 2002).
Beginning with the Mio-Pliocene radiation, the postcranial skeleton of Leporidae acquires more cursorial adaptations. Overall, the limb bones become slenderer and the tibiofibula and foot complex (including also tarsal elements) elongate (e.g., Fostowicz-Frelik, 2007). Studies of Neogene leporid postcrania based on large samples and/or complete specimens are relatively uncommon. Most studies focus on cursorial and fossorial adaptations, for example, (1) a partial skeleton of Trischizolagus (early Pliocene, Moldova; Averianov, 1995) suggests it was less cursorial than Hypolagus and was a strong digger, although not as fossorial as Oryctolagus; and (2) a large sample of Serengetilagus (based on forelimb anatomy; hindlimb not yet studied) ally this genus with smaller, relatively less cursorial leporids such as Oryctolagus and suggest it may have been semi-fossorial (early Pliocene, Tanzania; Winkler et al., 2016). As an example, from Ochotonidae, Dawson (1969) described the osteology of an abundant, albeit geologically younger (Quaternary, ca. 2.6 Ma to 200 BP) species Prolagus sardus from collections primarily from Corsica and Sardinia. Dawson concluded that the species “was probably not suited for speed over any great distance but was probably fairly adept at digging and well adapted for climbing and scrambling…” (Dawson, 1969, p. 187).
Phylogenetic Placement of Lagomorpha
The placement of Lagomorpha within the larger mammalian clade had been problematic for over a century (see Kraatz et al., 2010 for a review) due to an incomplete fossil record that did not include important stem lagomorphs. The earliest molecular phylogeny based on the eye lens protein alpha-crystallin revealed a close phylogenetic relationship of rabbits to primates (de Jung et al., 1977). That work also showed that rodents and lagomorphs form a supraordinal group (Glires) based on the interphotoreceptor retinoid binding protein (IRBP) (de Jung et al., 1977; Stanhope et al., 1992, 1996). New fossil discoveries such as the primitive rodent Tribosphenomys from strata of transitional Paleocene-Eocene age in Inner Mongolia (China) and Mongolia (Meng et al., 1994; Asher et al., 2005) began to support the close relationship between lagomorphs and rodents as cohort Glires. However, 91 orthologous protein sequences supported Lagomorpha as more closely related to Primates and Scandentia (treeshrews) than they are to rodents (Graur et al., 1996). The monophyly of a Glires clade was supported by complete mitochondrial genomes (Lin et al., 2002) and was consistent with the result of three nuclear studies, which included the von Willebrand Factor, an interphotoreceptor retinoid-binding protein, and an Alpha 2B adrenergic receptor (Huchon et al., 2002). Subsequently, a phylogenetic reconstruction based on 18 homologous gene segments confirmed Glires as a sister taxon to primates, colugos and treeshrews (Douady and Douzery, 2003). Analyses based on eight housekeeping genes further confirmed the monophyly of Glires (Kullberg et al., 2006). This was further evidenced by genome level data, including the monophyly of Glires, their close relationship with Primates, Scandentia and Dermoptera; and that all these taxa together formed the clade of Euarchontoglires (=Supraprimates) (Kumar et al., 2013; Foley et al., 2016; Esselstyn et al., 2017; Upham et al., 2019; Genereux et al., 2020).
Phylogenetic Relationships Within Lagomorpha
Early studies of morphological and ecological traits of extant lagomorphs resulted in different phylogenetic hypotheses (Dawson, 1981; Stoner et al., 2003). Considerable homoplasy in the morphology of leporid species was identified by Corbet (1983) after examining 21 morphological characteristics for 22 leporid species. More recent morphometric studies of lagomorphs have also found a high degree of homoplasy, low phylogenetic signal, and adaptive divergence in skull shape (Ge et al., 2015; Kraatz and Sherratt, 2016; Feijó et al., 2020). These studies highlight the difficulties in reconstructing a robust phylogeny for lagomorphs, particularly at the intergeneric level, by using morphological data. Relationships of extant genera were reconstructed based on the combined matrix of five nuclear and two mitochondrial DNA fragments: Ochotona is the earliest diverging taxon, which represents a relict genus of Ochotonidae; Nesolagus, Poelagus, and Pronolagus form an early diverging monophyletic clade within Leporidae. Romerolagus, Lepus, Sylvilagus, Brachylagus, Bunolagus, Oryctolagus, Caprolagus, and Pentalagus form another clade of Leporidae (Matthee et al., 2004; Robinson and Matthee, 2005). The general phylogenetic structure of the tree was supported by genomic orthologous retroposon insertion sites (Kriegs et al., 2010).
Within Lagomorpha, Lepus and Ochotona represent the most speciose extant genera. Molecular phylogenies within each of these genera have been extensively studied. The early studies are generally based on a single locus mitochondrial DNA marker, cytochrome b (CYTB) (Yu et al., 1996; Halanych et al., 1999; Niu et al., 2004). Five major species groups within Ochotona were recognized: the northern group, the surrounding Qinghai-Tibet Plateau group, the Qinghai-Tibet Plateau group, the Huanghe group, and the Central Asia group (Niu et al., 2004; Lanier and Olson, 2009). Subsequently, more genes were included, for example, the dataset of CYTB 12S, ND4, and the control region of the mitochondrial genome revealed the Chinese hare (Lepus sinensis) is not a monophyletic group, with three species groups recognized within Lepus: North America species group, South Africa species group and the Eurasia species group (Wu et al., 2005; Liu et al., 2011). Recent studies based on exome of the whole genome supported five subgenera of extant Ochotona: Alienauroa, Conothoa, Ochotona, Lagotona, and Pika, with divergence time and phylogeographic analyses inferring the last common ancestor of extant pikas first occurred in the middle Miocene, approximately 14 Ma (Wang et al., 2020).
Mito-nuclear discordance was shown in Lepus (Kinoshita et al., 2019) and Ochotona (Lissovsky et al., 2019), which could be the result of incomplete lineage sorting, sex-biased dispersal, asymmetrical introgression, natural selection, or Wolbachia-mediated genetic sweeps. The genome of four lagomorph species (O. cuniculus, L. timidus, L. americanus, and O. princeps) has been sequenced and annotated (Marques et al., 2020). These data provide references for more deep level studies in exploring the morphology, behavior, as well as population genetics of lagomorphs. However, more novel sampling is still needed for a complete phylogenomic analyses of Lagomorpha. Moreover, integrating data from fossils with extant species probably will provide a more comprehensive overview on the evolutionary history of lagomorphs.
The Fossil Record
Lagomorphs of modern aspect are known in the fossil record since the Early Eocene (ca. 52 Ma) of China (Li et al., 2007; Wang et al., 2010) and Mongolia (Lopatin and Averianov, 2008). The Asian record of Lagomorpha precedes that of North America by over 10 million years, and that of Europe by almost 20 Ma. Asia is considered the diversification center for the Duplicidentata, treated as a more inclusive group including crown lagomorphs and species more closely related to extant lagomorphs than rodents. Many of these earliest species are characterized by a double set of the upper and lower incisors and are referred to the ancestral Mimotonidae (Meng and Wyss, 2001; Fostowicz-Frelik et al., 2015b; Fostowicz-Frelik, 2017), an extinct fossil group restricted to China, Mongolia, and Kyrgyzstan (Li, 1977; Li and Ting, 1985; Averianov, 1994; Asher et al., 2005; Li C. K. et al., 2016; Fostowicz-Frelik, 2020). The mimotonids are a paraphyletic group with two distinct lineages: the small Paleocene mimotonids (Li, 1977; Li C. K. et al., 2016; Fostowicz-Frelik, 2020) and the large Eocene forms (Bohlin, 1951; Averianov, 1994; Meng et al., 2004; Asher et al., 2005; Fostowicz-Frelik et al., 2015b). One of the large forms, Mimolagus, likely survived to the Eocene–Oligocene boundary (Bohlin, 1951; see also Zhang and Wang, 2016) and was the terminal representative of the mimotonids (Fostowicz-Frelik et al., 2015b). Although Lagomorpha and Mimotonidae share many similarities in dental structure, most of these characters are plesiomorphic; thus, none of the mimotonids could be unquestionably named the direct ancestor of lagomorphs. All the Eocene lagomorph taxa and a substantial part of the Oligocene species are regarded as stem lagomorphs, although they frequently show similarities to either of the crown groups (Leporidae and Ochotonidae, see Fostowicz-Frelik and Meng, 2013).
The earliest findings of non-mimotonid stem lagomorphs come from the latest Early Eocene of Asia: Dawsonolagus from Inner Mongolia, China (Li et al., 2007), and Arnebolagus from Mongolia and Kyrgyzstan (Averianov and Lopatin, 2005; Lopatin and Averianov, 2008, 2020). By the Middle Eocene, China and Kyrgyzstan witnessed the first lagomorph diversification, yielding multiple genera (Li, 1965; Tong, 1997; Averianov and Lopatin, 2005; Meng et al., 2005; Fostowicz-Frelik et al., 2012, 2015a; Fostowicz-Frelik and Li, 2014; Li Q. et al., 2016). By the end of the Eocene, the lagomorph fauna of Asia was enriched, in particular, by Desmatolagus (Meng et al., 2005), a key lagomorph genus for the Oligocene in Asia (Erbajeva and Daxner-Höck, 2014).
Asian Eocene lagomorphs were very small, with an estimated body mass under 150 g (Fostowicz-Frelik et al., 2015b). Starting from the Middle Eocene, Asian lagomorphs doubled in size, but even then, most of the Paleogene genera did not exceed the size of a large Ochotona (ca. 250 g). With the beginning of the Oligocene, the Desmatolagus lineage became diverse, abundant, and widespread throughout Central Asia (Sych, 1975; Huang, 1987; Wang, 2007), surviving until the Miocene, and possibly entering Europe (Early Oligocene, Vianey-Liaud and Lebrun, 2013) and North America (Late Oligocene; Dawson, 2008). Along with Desmatolagus, in the late Early/Middle Oligocene, the first plausible representatives of crown lagomorphs appear in Asia: Sinolagomys (an early ochotonid from China and Mongolia; see Erbajeva et al., 2017) and Ordolagus (probably an early leporid from China; Bohlin, 1942).
In North America, the lagomorph fossil record starts in the late Middle Eocene (ca. 42 Ma; see Dawson, 2008). Two genera, Mytonolagus and Procaprolagus, are known from this period and likely represent two different immigrations from Asia (Dawson, 2008). There is a significant increase in diversity in the Lagomorpha of North America beginning in the Late Eocene: this includes genera with true hypselodont cheek teeth such as Chadrolagus (Gawne, 1978; Fostowicz-Frelik, 2013) and the first representatives of Palaeolagus and Megalagus (Dawson, 1958, 2008). At the Eocene-Oligocene boundary (EOB), a turnover in the lagomorph fauna is observed, defined by a shift from unilateral hypsodonty (i.e., high-crowned, evergrowing lingual sided and low-crowned buccal sided cheek teeth) toward a hypselodont condition in their cheek teeth (‘full’ hypsodonty). Among Megalagus and Palaeolagus, the unilaterally hypsodont species went extinct at the EOB and were replaced by species either with greatly reduced buccal roots, or fully developed hypselodont cheek teeth. Litolagus, with its advanced cranial morphology, may represent either crown Leporidae (see Fostowicz-Frelik, 2013) or an advanced stem taxon. Later during the Early Oligocene, other hypselodont species appear, for example, Palaeolagus burkei may be closely related to Litolagus or it may have convergent traits in dentition and bulla structure, which could be a result of adaptations to more open habitats of the North American plains. All lagomorph lineages that originated during the Eocene-to-Oligocene interval in North America went extinct by the Early Miocene (Dawson, 2008).
In Europe, the earliest lagomorphs are known from the Early Oligocene of France (Ephemerolagus nievae; Vianey-Liaud and Lebrun, 2013) and Germany (Shamolagus franconicus; Heissig and Schmidt-Kittler, 1975, 1976). The remains are scarce, and their appearance is limited only to the type localities, but these genera clearly represent two distinct lineages. Lagomorph lineages reappearing in Europe by the end of the Oligocene (Tobien, 1974, 1975) are regarded as either primitive ochotonids (McKenna, 1982) or as stem line representatives (Fostowicz-Frelik and Meng, 2013). These lineages persist in Europe from the Late Oligocene (Fostowicz-Frelik, 2016) until the Early Miocene (Tobien, 1974).
The Early to Middle Miocene (beginning ca. 23 Ma) is characterized by the last records of the stem lagomorphs and the worldwide radiation of the Ochotonidae (Dawson, 2008; Ge et al., 2013). The Early Miocene record of stem Lagomorpha consists mostly of derived Desmatolagus (Lopatin, 1998; Wang et al., 2009) and Asian representatives of Amphilagus, a Late Oligocene-Early Miocene genus from Europe, which has been reported recently also from Mongolia and Siberia (Erbajeva, 2013; Erbajeva et al., 2016). In North America, the earliest Miocene marks the last appearance of taxa such as Megalagus and Palaeolagus (Dawson, 2008). In Europe a plethora of Ochotonidae appeared in the Early Miocene, for example Alloptox and Prolagus (Tobien, 1974, 1975; López Martínez, 2001). In the Early and Middle Miocene taxa such as these existed alongside the stem lineages, which went extinct no later than the Middle Miocene (Tobien, 1974; Fostowicz-Frelik et al., 2012). Prolagus, first reported from the Early Miocene, was the most speciose and long-lived lineage of the European ochotonids and the last species, P. sardus, survived in the Mediterranean until historic times (Lopez-Martinez, 2008). Simultaneously, starting from the Middle Miocene, a lineage leading to extant Ochotona, the only surviving member of the Ochotonidae, arose in Central Asia (Wang et al., 2009; Fostowicz-Frelik and Frelik, 2010): this lineage also flourished in Asia during the Pliocene.
The earliest known ochotonids from North America are from near the Oligocene-Miocene boundary: all these early immigrants went extinct not later than ca. 9 Ma (Dawson, 2008). Ochotona was first reported in North America in the Late Miocene: this genus was geographically and taxonomically diverse during the Late Miocene-Pliocene in the Northern Hemisphere (Erbajeva et al., 2015). The genus decreased in diversity and relative abundance beginning in the Pleistocene (ca. 2.6 Ma; Erbajeva et al., 2015).
The earliest lagomorphs to reach Africa were representatives of the Asian sinolagomyine ochotonids, which dispersed into Africa as far as southern Africa in the Early Miocene (Winkler and Avery, 2010). Extinction of the African sinolagomyines by the Middle Miocene was coincident with the global extinction of archaic ochotonids by the end of the Middle Miocene (Erbajeva et al., 2015). The only post Middle Miocene reports of ochotonids from Africa are Prolagus, reported from the Late Miocene to Early Pleistocene of northern Africa (López Martínez, 2001; Winkler and Avery, 2010). In contrast to the ochotonids, Ge et al. (2013) noted that the diversity of the Leporidae was relatively modest during much of the Miocene, increasing around the Miocene-Pliocene transition, and with high diversity continuing into the Pliocene and Pleistocene.
The earliest record of leporids in Africa is in the Late Miocene (Winkler and Avery, 2010) as part of a geographically widespread and relatively abrupt dispersal of leporids at ca. 8 Ma that Flynn et al. (2014) called the Leporid Datum. Leporids dispersed from North America to northern Asia, spread throughout Eurasia, and entered Southern Asia (by 7.4 Ma) and Africa (ca. 7 Ma) (Flynn et al., 2014). Ge et al. (2013) correlated the geographic dispersal and increase in diversity of the leporids around the Late Miocene (and the opposite response of the ochotonids) with a period of global cooling and drying, and the expansion and diversification of C4 plants (at the expense of the C3 plants) in tropical and temperate areas.
Key Functional Genes of Lagomorphs
Wild populations of lagomorphs are greatly affected by two diseases, rabbit hemorrhagic disease and myxomatosis. The genes relating to the immune system and these diseases are well studied. These are, for example, interleukins, chemokines and chemokine receptors, Toll-like receptors, antiviral proteins (RIG-I and Trim5), and the genes encoding fucosyltransferases that are utilized by rabbit hemorrhagic disease virus as a portal for invading host respiratory and gut epithelial cells (Pinheiro et al., 2016). Fourteen IgA (immunoglobulin A) subclasses have been identified in O. cuniculus, eleven of which are expressed. In contrast, most other mammals have only one IgA, or in the case of hominoids, two IgA subclasses (Pinheiro et al., 2018). VHn genes are a conserved ancestral polymorphism that has been maintained in the leporid genome and being used for the generation of VDJ rearrangements by both modern Lepus and Oryctolagus (Pinheiro et al., 2019). Toll-like receptors (TLRs) are one of the first lines of defense against pathogens and are crucial for triggering an appropriate immune response: strong selection of the TLR2 coding region among the Lagomorpha suggests an evolutionary history that differs from other mammals (Neves et al., 2019). A high level of variation in the tripartite motif-containing protein 5 alpha (TRIM5) PRYSPRY domain of Lagomorpha species that belong to the same genus was believed to restrict retroviral infections (Águeda-Pinto et al., 2019). Recent study revealed the winter coat color polymorphism of snowshoe hares was associated to the genomic region of the pigmentation gene Agouti (Giska et al., 2019; Jones et al., 2020). Genetic variation at Agouti clustered by winter coat color occurs across multiple hare and jackrabbit species (Jones et al., 2018).
Hybridization in Lagomorpha
Hybridization may accelerate speciation via adaptive introgression or cause near-instantaneous speciation by allopolyploidization (Abbott et al., 2013). There are many articles referring to the hybridization, gene flow or reticulate evolution of lagomorphs (Table 1). Previous studies reported hybridization occurred within Lepus, Oryctolagus, and Ochotona usually based on single, multilocus DNA markers, and microsatellite loci (Chapman and Morgan, 1973; Wu et al., 2011; Koju et al., 2017). In some cases, selective advantages of hybrid forms to special climate condition in the contact zone and competitive exclusion of parental forms causes hybrid superiority over parental species (Mohammadi et al., 2020) because enhanced reproductive success may be due to the selective advantages of new combinations of mito-nuclear packages.
In addition, transitional phenotype of hybrids and introgressions also encounter traditional taxonomy with confusion in hybrid zones while reticulate and mosaic evolution of the genome and incomplete lineage sorting especially within nuclear loci also make application of new molecular tools such as DNA barcoding for identification of species useless. Plausible conspecificity have been raised from lack of morphological diagnostic characters and low genetic divergence in phylogenetic reconstructions based on some few nuclear loci (Liu et al., 2011). There are still gaps in sampling from type localities of some taxa (e.g., Lepus tibetanus pamirensis; type locality: near Lake Sarui-Kul, Pamir Mountains) and gaps for understanding the intraspecific genetic diversity (e.g., in Lepus saxatilis from Africa, in Lepus melainus and all other kinds of Manchurian hares). The taxon przewalskii is still controversial and the taxonomic status of centrasiaticus has not been resolved due to its morphological similarities to Lepus oiostolus, and its morphometric (Cheng et al., 2012) and molecular affinity to L. tolai (Wu et al., 2011; Smith et al., 2018). Possible paraphyly of some taxa such as L. timidus and Lepus tolai in China (Wu et al., 2005; Shan et al., 2020); Lepus capensis s.l. in Africa (Lado et al., 2016) and L. timidus from northern Europe, Siberia, and Fennoscandian regions (Waltari and Cook, 2005) add to the complexity of the taxonomic status of some of the members of the genus Lepus and essential need for revision based on complete genome phylogenetic analyses. There are many reports of hybridization between different species like between L. tolai, and L. timidus with L. sinensis, from L. sinensis into L. mandshuricus (Liu et al., 2011), between L. tolai and L. yarkandensis (Wu et al., 2011), L. timidus into L. granatensis and L. europaeus (Alves et al., 2003), from L. europaeus into L. tolai (Mohammadi et al., 2020). Sharing of the same haplotypes between two different species and some cases of hybridization and introgression between sister taxa have been also reported within pikas [e.g., between Ochotona cansus and Ochotona curzoniae (Koju et al., 2017), Ochotona dauurica and O. cansus (Lissovsky et al., 2019), O. curzoniae and Ochotona nubrica (Yu et al., 2000; Niu et al., 2004; Lissovsky, 2014; Lissovsky et al., 2019)]. Moreover, lack of type specimens and the probable presence of hybrid forms even in the type localities, which makes molecular comparisons doubtful (e.g., for L. tolai; see Mohammadi et al., 2020) and have raised even further questions concerning the taxonomy and evolutionary relationships between taxa. More comprehensive studies are needed to address taxonomic challenges remaining around the North American white-tailed jackrabbit Lepus townsendii, Lepus corsicanus from Italy, and Lepus castroviejoi from the Iberian Peninsula.
The vast variety of introgression and evidence of hybridization between two species in areas of sympatry and parapatry (e.g., between L. europaeus and L. tolai in Iran; L. europaeus and L. timidus in Sweden; L. tolai and L. yarkandensis in Tarim Basin, China) and the lack of evidence of hybridization in other cases of geographical sympatry (e.g., between L. europaeus and L. tolai in Kazakhstan; between L. tibetanus, L. oiostolus, and L. tolai in China; L. yarkandensis and L. timidus also in China) have suggested the genus Lepus as a good natural model for studying and tracing hybridization and the speciation process and also highlights the insufficient taxonomic knowledge to identify many of the taxa indicated as hybridized in scientific literature.
Domestication of Leporidae
The domesticated rabbit is derived from O. cuniculus and has its history in early European cultures (Clutton-Brock, 1989). While it is hypothesized that the Romans spread wild rabbits out of the native Iberian Peninsula to much of Europe and British Isles, they did not attempt to domesticate it. Several authors recounting the history of rabbit domestication place the origin with French Medieval monks (Clutton-Brock, 1989; Naff and Craig, 2012), where rabbits were kept in hutches or walled gardens to be fattened up for consumption, and thus selectively bred to increase body size. The morphological diversity among the ∼50 breeds of the domesticated rabbit known today is driven by body size (e.g., dwarf and giant forms), thus many differences in morphological features are likely a result of allometry, the associated shape changes with size, and heterochrony, the changes in timing of development (e.g., Fiorello and German, 1997; Sánchez-Villagra et al., 2017). Studies of morphological variation in companion and laboratory rabbits are predominantly focused on pathological and skeletal abnormalities resulting from their continual tooth growth (e.g., Okuda et al., 2007; Böhmer and Böhmer, 2017; Parés-Casanova and Cabello, 2020).
Lagomorph Development
Our knowledge of lagomorph development is based primarily on the common laboratory rabbit, O. cuniculus, because this species is easy to keep and breed and therefore early ontogenetic stages are readily available. O. cuniculus is an induced ovulator (Boussit, 1989; Delaney et al., 2018). Sexual differentiation is established on the 16th day of embryonic development, and oogenesis continues for about 2 weeks after birth (Mauleon, 1967; Kennelly et al., 1970). Graafian follicles appear in the New Zealand breed at 12 weeks of age. Ovulation occurs between 10 and 12 h after mating and the peak of fecundity is observed between 12 and 15 h post coitum (pc) (Harper, 1961; Thibault, 1967; Foote and Carney, 2000). Based on the observations of Lopez-Bejar (1995), embryos have completed their first cell cycle at 26 h pc, then continue to divide to reach the 4-cell stage at 26–32 h pc, followed by 8-cell (32–40 h pc), 16-cell (40–47 h pc), morula (47–68 h pc), and blastocyst (68–76 h pc) stages. The embryo begins to implant 7 days after fertilization (DeSesso, 1996). The blastocyst loses its zona pellucida, which is replaced by layers of glycoproteins whose adhesive properties play an important role in implantation (Alliston and Pardee, 1973). The ectoblast covers a deep layer or endoblast. A medium or mesoblast layer is isolated between the two previous layers. The embryo begins to lie down on the 8th day of gestation; then on the 11th day the head becomes dominant in size and the limbs lengthen. From the 19th day (end of organogenesis), the limbs are well formed, and the muzzle lengthens. From 12th day of gestation, development of the bi-discoid and hemochorial placenta will ensure the growth and development of the fetus until parturition (Rodriguez et al., 1985). The weight of the young rabbits does not change much until the 16th day but then increases very quickly: between 24th and 30th day, the rabbit fetus multiplies its weight by six (Bruce and Abdul-Karim, 1973). Gestation is from 30 to 33 days.
Understanding the development of the mammalian cranium requires the investigation from early prenatal to adult stages (Gaupp, 1906; Novacek, 1993; Maier and Ruf, 2014). These data provide the ultimate baseline for character polarization and a deeper understanding of ontogenetic transformations into adult stages and thus, significantly contribute to comparative anatomical, morphofunctional, systematic and evolutionary studies (e.g., Maier and Ruf, 2014; Sánchez-Villagra and Forasiepi, 2017; Ruf, 2020). To date models of cranial development in Lagomorpha are still mainly based on Oryctolagus cuniculus (e.g., Voit, 1909; de Beer and Woodger, 1930; Frick and Heckmann, 1955; Hoyte, 1961) because access to ontogenetic series is easy. Only a few other species are known by pre- or perinatal stages, although their cranial development has not been studied in as much detail. These are: L. capensis (Eloff, 1950), Ochotona rufescens and Ochotona roylei (Insom et al., 1990), and Ochotona sp. (Frahnert, 1998). However, all these studies comprise single or very few stages that were prepared as histological serial sections. Postnatal ontogeny of the lagomorph cranium has been mainly investigated based on osteological features, for instance, skull size and shape changes in O. curzoniae, L. oiostolus, and L. capensis (Lu, 2003; Zhang and Ge, 2014), growth of the ear capsule in O. cuniculus (Hoyte, 1961). Regarding cranial development, Lagomorpha, and especially Leporidae, can be the ideal model organism because they comprise different modes of reproduction (altricial, precocial) that help to elucidate developmental and evolutionary constraints in lagomorph evolution. The ontogenetic transformation of cranial features in altricial versus precocial species provides key features to character polarization and transformation serving as baseline for the understanding of systematics, morphofunction, and evolution of the respective species and clades. This has been attempted recently by the study of perinatal dental eruption in selected Glires (including O. cuniculus and L. europaeus) or the comparison of chondrocranial transformations in altricial and precocial Muroidea (Ruf, 2020; Ruf et al., 2020).
Comprehensive studies of the cranial development in selected lagomorph species that include ontogenetic series spanning from fetal to adult are still pending, although sufficient material of selected species is certainly available in scientific collections. This approach is essential to understand cranial characters and adult patterns, as demonstrated by a recent study on the auditory ossicles in lagomorphs (Maier et al., 2018). Based on both histological serial sections of perinatal stages and μCT scans of adult skulls of Ochotonidae and Leporidae, Maier et al. (2018) revealed a family-specific pattern of the processus anterior and processus internus praearticularis of the malleus. The perinatal stages provide insight into the development of the two very different adult patterns that correlate with adaptations in sound transmission and allow for refining previous functional classifications of mammalian auditory ossicles (Maier et al., 2018).
New Methods in Assessing Development
The cranial anatomy and development of prenatal to early postnatal stages in mammals traditionally have been investigated using histological serial sectioning (Hall, 2005). Although this method is destructive and time consuming it allows the detailed study of hard and soft tissue structures and has led to important observations regarding the development of soft tissue (e.g., Maier et al., 2002; Ruf et al., 2009). Advances in modern imaging techniques such as μCT, allow for non-destructive investigation of any ontogenetic stage, as well as rare, collection specimens. Promising new diffusion based methods that increase the radio-opacity (and thus contrast) of soft tissues in specimens prior to μCT scanning are becoming more widespread and represent a frontier in the study of development in a wide variety of taxonomic groups (Metscher, 2009a, b; Gignac et al., 2016). Phosphotungstic acid and inorganic iodine are the two stains that are becoming increasingly common research tools; they are relatively easy to handle and produce high-contrast X-ray images of many soft tissues. Iodine (IKI and I2E) staining followed by μCT scanning works particularly well with mammals, including lagomorphs (Racicot and Ruf, 2020; Figure 3) and also is reversible using simple destaining methods. Specimens still may be processed for histological staining and sectioning or other methods after μCT scanning, and thus provide a record of their original internal and external three-dimensional geometries prior to employing subsequent destructive methods.
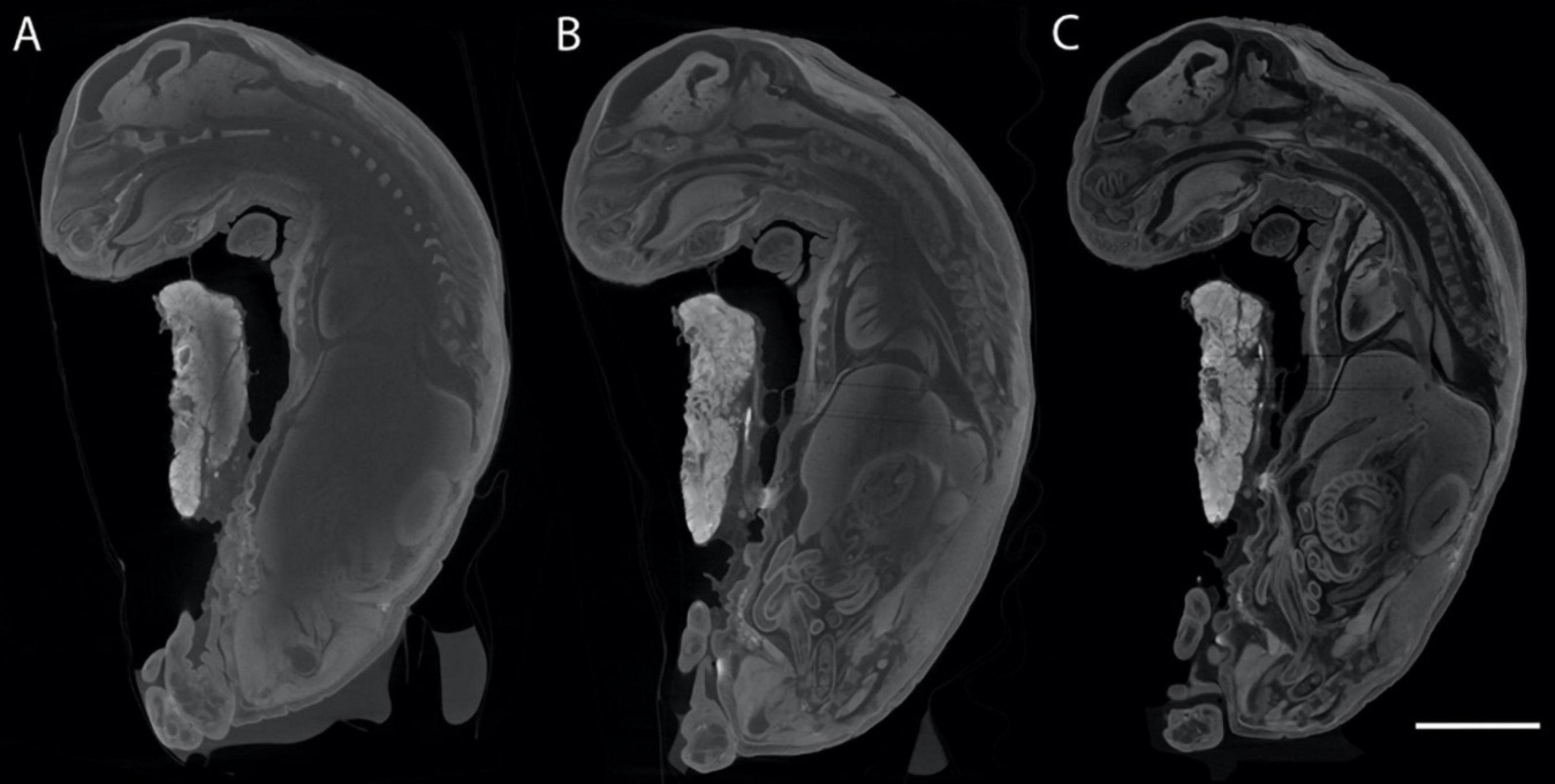
Figure 3. Sagittal μCT slices taken from generally the same area of the same O. cuniculus specimen (Racicot and Ruf, 2020), stained for different lengths of time to show increasing contrast imparted by the Lugol’s iodine stain. (A) Stain penetration after 5 weeks and 2 days, (B) penetration after 7 weeks, (C) stain penetration after 11 weeks and 2 days. Scale bar of 10 mm applies to all specimens.
While μCT scanning has become a standard method for paleontologists, biologists, and museum researchers (e.g., Racicot, 2017), using staining to increase radio-opacity before μCT scanning still is a relatively new approach. Some general guidelines and examples of success with iodine staining and scanning methods are described in the literature (summarized in Metscher, 2009a, b; Gignac et al., 2016). Iodine provides clear contrast between neural and other tissues because its inner shell electron binding energy is similar to lower energy X-rays used in scans and often is used in medical contexts.
The most conservative methodology for rare museum specimens has been exemplified by recent work on cetaceans (Lanzetti et al., 2018) and tested with a prenatal stage of a lagomorph (Figure 3). Specimens that are preserved in formalin may be directly placed either in pre-prepared or self-prepared ∼1% Lugol’s iodine solution (e.g., Figure 3). For specimens preserved in 70% alcohol, metal iodide is mixed first in the highest alcohol concentration possible (96–100%), then diluted to 70% alcohol with iodine before beginning staining. Rotating or moving the specimens either constantly or daily while staining is recommended because the stain can settle.
Other staining methods have been employed but may not work well with larger specimens. Phosphotungstic acid can be used effectively, particularly after fixation with Bouin’s solution, but penetration times are slower than iodine methods, and it is unclear whether this method is reversible (Metscher, 2009a, b). It has been suggested that specimens fixed in Bouin’s solution and preserved in 70% alcohol can be stained with I2E before μCT scanning (Metscher, 2009b) for effective increase in contrast. After the staining and scanning process is completed, specimens can be destained using 3% sodium thiosulfate dissolved in deionized water (for formalin specimens) or 70% ethanol (for ethanol specimens). The specimen subsequently can be used for future research, including re-staining and/or sectioning as desired. It is recommended to note in the documentation associated with the specimen that the specimen has been stained and μCT scanned previously. Some workers have noted that DNA perhaps should not be extracted from specimens after this procedure (Gignac et al., 2016) because the impact of staining on DNA analyses has not been explored: experiments along these lines thus may be interesting. μCT scanning in combination with iodine staining represents a promising frontier in studying the development and ontogeny of lagomorphs, particularly in rare species/museum specimens for which destructive methods are unattractive options. Maintaining the 3D geometry of soft tissues, while also ‘remotely’ observing these tissues with high contrast will increase the natural history value of the specimens by providing a permanent digital record that can also be used in educational contexts.
Functional Morphology
Leporids are characterized by increased cursoriality, in general; pikas are the least cursorial and jackrabbits the most cursorial, with rabbits and cottontails (e.g., Sylvilagus) occupying an intermediate position (Camp and Borell, 1937; Gambaryan, 1974). A small number of previous studies have discussed the gradation in cursoriality among lagomorphs and sought to identify morphological correlates of this behavioral cline (Camp and Borell, 1937; Gambaryan, 1974; Bramble, 1989; Kraatz and Sherratt, 2016). Among lagomorphs, leporids are perhaps best known for their gait, in which maximum running speeds of some species match the upper limit of racehorses and greyhounds; a speed unknown for any other mammal of leporid size. Leporid speeds vary from 40 km/h (11 m/s) in Sylvilagus through 56 km/h (16 m/s) in O. cuniculus and up to 72 km/h (20 m/s) in L. europaeus and Lepus alleni (Garland, 1983). While leaping abilities are common among most leporid lineages, they are also known to be facultatively semiaquatic, scansorial, fossorial, or exhibit a more generalized, non-hopping form of locomotion (Chapman and Flux, 1990). The most comprehensive framework for understanding lagomorph locomotion and locomotor apparatus in an evolutionary perspective was proposed by Gambaryan (1974), who suggested that lagomorphs originated as talus-dwellers and, therefore, regarded ochotonids as living fossils (Figure 4). The coupled half-bound action of the hindlimbs in lagomorphs is suggested to be the adaptative remnant of leaping from rock to rock. Among the variety of quadrupedal asymmetrical gaits in mammals, Gambaryan (1974) distinguishes two main categories: (1) true gallops, including the lagomorph half-bound and (2) ‘the primitive ricochet.’ The latter should not be confused with bipedal ricochet (i.e., proper hopping) of kangaroos and jerboas. According to Gambaryan (1974), the primitive ricochet is retained by many poorly running mammals, including most of the rodents.
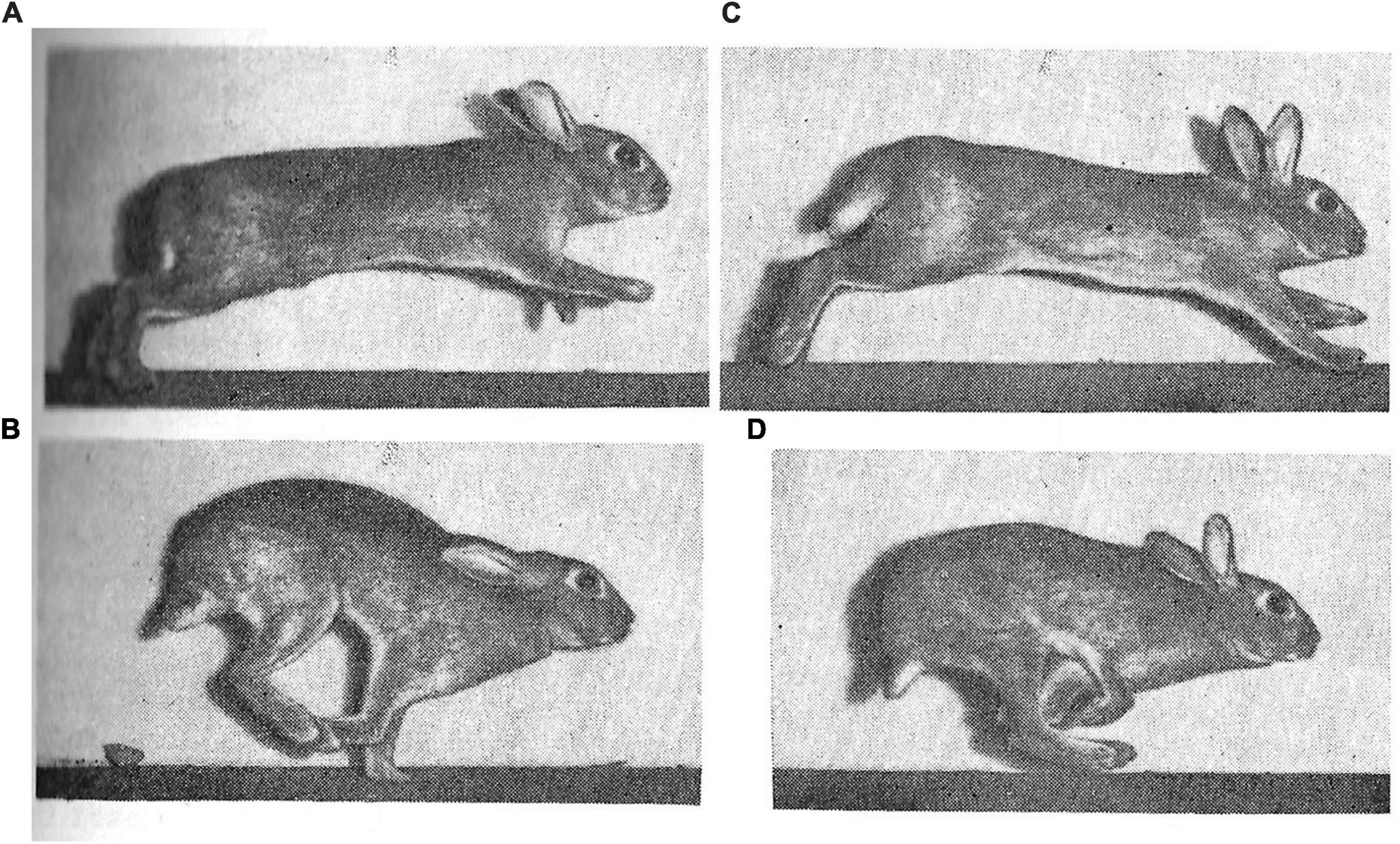
Figure 4. Stages of wild rabbit (O. cuniculus) gait as captured and described by Gambaryan (1974), including: (A) support on hindlimbs; (B) extended flight; (C) support on forelimb; and (D) crossed flight.
The main difference between the true gallop and the primitive ricochet is the pattern of hindlimb motion during the extended suspension. In the primitive ricochet hindlimbs start protraction immediately as they finish their thrust and lose contact with the ground. In contrast, in the true gallop, there is a considerable delay in the hindlimb protraction where hindlimbs are held in retracted position from the end of their contact phase and throughout the extended suspension. The hindlimbs protract in gallop (e.g., lagomorph half-bound) when the forelimbs are landed; the forward swing of the hindquarters is much faster than in the primitive ricochet. According to Gambaryan (1974), the advantage of the hindlimb delay is the ability of an animal to arrive at the landing point more precisely when the hindlimbs are held fixed during a leap. Landing precision is crucial on irregular substrates such as tree branches and rocks, but also, the hindlimb delay would likely be advantageous while covering obstacles at high speeds. Gambaryan (1974) summarized lagomorph locomotor patterns as a dorsomobile-metalocomotor type. While a metalocomotor type implies the prevalence of the hindlimbs, dorsomobile implies an additional active usage of the spine; more exactly, the use of lumbar region. Lagomorphs not only actively extend their spine with hindlimb thrust, but also flex their spine when the forelimbs are landed, and the hindquarters need to be rapidly protracted.
Recording Lagomorph Gaits
The first image sequences of lagomorph locomotion were recorded as early as in 1893–1894 by Étienne-Jules Marey, which capture a very slow half-bound of the white domestic rabbit (O. cuniculus)1. However, images from those movies were not included as illustrations in his subsequent publications (Marey, 1894, 1901). This is likely why Pablo Magne de la Croix, an Argentinean Frenchman who was among the first to study the evolution of quadrupedal gaits, had an incorrect impression on the rabbit’s gait (Magne de la Croix, 1928, 1933, 1936); he only depicted an extended (centrifugal) suspension, and no gathered (centripetal) suspension stage in the locomotor cycle of the rabbit. The former is characterized by the straightened spine, retracted hindlimbs and protracted forelimbs, while in the latter the spine is flexed, the hindlimbs are protracted and cross the retracted forelimbs. In rabbits, with speed growth, there is at first no suspension in the locomotor cycle, then the gathered suspension appears (missed by Magne de la Croix), and finally the extended suspension is added (not recorded by Marey). Both stages of suspension were recorded by Gambaryan (1974) in the wild European rabbit 80 years after Marey’s first observations. Also, Gambaryan’s (1974) work was the first publication representing the frames of cinematographic record of the running cycle of the Alpine pika (Ochotona alpina). He noted that in pika the gathered suspension is almost absent, but the extended stage is prolonged to ensure leaping from stone to stone in their usual rocky habitat.
After Marey (1894, 1901) and Gambaryan (1974), there appeared in scientific publications a few additional illustrations of running lagomorphs. Dimery (1985) filmed wild rabbits at a high frequency (300 frames per second) but depicted only the outlines of the hindlimb movements. Bramble (1989) depicted the fast-running cycle of Lepus californicus, showing both the gathered and the extended suspension and filmed at 200 fps. Simons (1999) depicted the fast-running cycle of the domestic rabbit with both suspension stages filmed at 100 fps in X-ray to visualize viscera movements. Bertram and Gutmann (2009) depicted the slow-running cycle of L. townsendii with only one suspension stage. Like in Marey’s faster rabbit, it appears after the forelimb thrust and, thus, corresponds to the gathered suspension. However, the forelimbs and hindlimbs are not really gathered under the body but remain parallel to each other like in a pronking (stotting) gait of gazelle or mara (Climaco das Chagas et al., 2019). Kuznetsov et al. (2017) published a video recording image sequence of a cornering maneuver of L. europaeus and also represented its gait diagram for maneuver and fast forward running. As to pikas, their half-bound gait was recorded due to successful keeping of a population of O. rufescens in Germany and appeared in a series of papers on locomotion of small mammals, with O. rufescens as a central object (Witte et al., 2002; Hackert et al., 2006; Schilling and Hackert, 2006). Both suspension stages were brief, so for pictures of faster running pikas one must return to Gambaryan (1974).
Limb Architecture
Camp and Borell (1937) were the first to compare the hindlimb bones and muscles of lagomorphs using a series of increasingly cursorial taxa: Ochotona, Sylvilagus, and Lepus. They note a tendency in this series in which muscle bellies become shorter, and tendons become longer. The first research of the entire postcranial locomotor apparatus of Mongolian species of pikas, aimed at ecological interpretation, was a dissertation subject of Cevegmid (1950), but has never been published. Klebanova et al. (1971) presented a detailed account of postcranial bone dimensions and muscle masses and attachments for six pika and five leporid species. Lammers and German (2002) attempted to find the specific features in the growth of limb bones of the rabbit and chinchilla, both of which use a half-bound gait. Williams et al. (2007a) quantified the hindlimb muscles and tendons of L. europaeus. They did not cite the early paper by Camp and Borell (1937) but explained their finding of increased development of tendons in the hare’s hindlimb as an adaption for elastic energy storage. The same authors (Williams et al., 2007b) published a similar analysis of the forelimb muscles of L. europaeus, showing that the tendons in the forelimb are less developed than in the hindlimb. This implies that the forelimbs have a smaller role in energy saving via elastic storage and recoil. Reese et al. (2013) compared limb bone proportions between the meadow-dwelling and talus-dwelling pikas and found differences that they qualitatively attributed to the different actions of digging in meadows versus leaping in rocks. Young et al. (2014) took the same series of increasing cursoriality represented by Ochotona, Sylvilagus, and Lepus, as was earlier studied by Camp and Borell (1937) and Fostowicz-Frelik (2007) and performed a morphometric analysis of fore- and hindlimb bones, examining relative length and robusticity. Their results suggest that with increasing cursoriality in lagomorphs, the proximal limb bones become more gracile (decrease in robustness), while the distal bones do not. They hypothesize that limb bone morphology of lagomorphs is indicative of a trade-off between locomotor economy (cursors requiring longer, gracile limbs) and the need to be fracture-resistant (i.e., more robust) in the distal regions involved in impact.
Locomotory Adaptations to Cornering
Although lagomorphs exhibit exceptional levels of maneuverability, there is only one scientific publication documenting this phenomenon (Kuznetsov et al., 2017). This study is based on field records of the L. europaeus chased by sighthounds. Hares’ stereotyped turn begins by a lateral kick of a forelimb against the ground, which initiates the turn to the opposite side of the kicking forelimb. Centripetal forces are produced by the forelimbs, and the braking forces by the hindlimbs. In contrast, the chasing dogs perform braking by the forelimbs and therefore, sometimes somersault overhead. The anterior and posterior halves of the hares’ trunk yaw and roll separately. Separate rolling employs axial rotation in the thoracic region of the spine. The morphological application of this study is the explanation of unique hypertrophy of the musculus subclavius in lagomorphs with its expansion over the whole scapula. This muscle became a specialized pronator of the scapula (there is no other efficient pronator of the scapula in mammals). Contracting together with the musculus serratus ventralis cervicis, it ensures centripetal force production by the forelimb and, first, the lateral kick at the touch-down of the forelimb initiating the maneuver. In leporids, the musculus subclavius comprises 1% of the total muscular mass of the limbs, while in rodents no more than 0.5% (Gambaryan, 1974). In pikas, it comprises 4% of the total muscular mass of the limbs. The musculus serratus ventralis cervicis, which assists the musculus subclavius in the lateral kick, comprises 1.5% of the total muscular mass of the limbs in leporids, and 2.3% in pikas. The greater relative mass of both muscles in pikas may highlight their elevated ability for sharp turning and smaller prevalence of the hindlimbs.
Locomotory Adaptations of the Spine
In the leporid vertebral skeleton, the most prominent structures related to the unique role of axial bending during the half-bound are the ventral spinous processes of the three anterior lumbar vertebrae and the posterior two or three thoracic vertebrae (Klebanova et al., 1971; Gambaryan, 1974). These processes provide an additional attachment site for the major lumbar flexors, which comprise 5–10% of the total muscular mass of the limbs in lagomorphs but never more than 5% in ungulates and carnivorans (Gambaryan, 1974). The antagonists extending the spine are equivalent to 30% of the total muscular mass of the limbs in leporids and 15% in pikas. In addition to the unique ventral spinous processes, leporids also show a set of skeletal features typical to other dorsomobile mammals. These are the elongate and anteriorly inclined transverse processes and short and anteriorly inclined dorsal spinous processes of lumbar vertebrae (Gambaryan, 1974; Jones et al., 2018).
Vertebral range of motion was studied in vitro in three lumbar joints (those between vertebrae L4–L7) of the rabbit (Grauer et al., 2000). It was found that each lumbar joint can be flexed ventrally by 12–15°, extended dorsally by 6°, bent laterally by 4–8°, and rotated axially to every side by about 1°. The prevalence of sagittal flexion and extension, as well as reduction of axial twisting of the lumbar region is expected given what is known about overall locomotor behavior in lagomorphs. In vivo spine movements in the sagittal plane were studied for pikas based on high-frequency filming in X-ray (Schilling and Hackert, 2006). Simons (1999) filmed rabbit running to study a visceral piston hypothesis according to which the breath in gallop is synchronized with the stages of locomotor cycle and with spine sagittal bending. The inertia of the viscera, and especially of the liver, are said to act as a pump for inhalation and exhalation, while the activity of the diaphragm and rib cage muscles might be virtually unnecessary. Simons (1999) falsified this hypothesis for the rabbit by showing inappropriate mutual phasing of locomotor and breathing cycles. Instead, an idea of pneumatic stabilization of the thorax was suggested.
Cranial Kinesis and Locomotion
Cranial kinesis is the ability of bones of the cranium to move relative to each other (as such, it is opposed to the mobility of visceral skeleton, e.g., jaw opening and movements of the middle ear ossicles). Cranial kinesis within leporids, unknown among other mammals, has been suggested to play an important role in locomotor behavior (Bramble, 1989), though the extent of this kinesis of leporid has not yet been fully characterized. In both ochotonids and leporids, ethmoid-orbital and otic-occipital part parts of the braincase are partially separated laterally by a piriform fenestra that passes subvertically along the posterior border of os alisphenoideum and (in leporids) os squamosum (Wible, 2007). Ventrally, the piriform fenestra comes into a transverse fissure which separates os basisphenoideum from os basioccipitale (in contrast to tight junction or even fusion of those two bones in other mammals). Notably, this is not a fetal, but an adult condition. Thus, the piriform fenestra and basisphenoid/basioccipital fissure serve as an intracranial joint, which Bramble (1989) suggests helps to dampen forces that occur during the locomotor cycle of lagomorphs, especially in high-speed hares. Due to soft tissue attachments across the intracranial joint, the large ears of hares may also act to support and reset the anterior (ethmoid-orbital) part of the cranium (Stott et al., 2010).
Bramble (1989) assumed that in steady quadrupedal running the forelimbs produce a significant braking effect, while the hindlimbs primarily accelerate the body. In fact, this was a common assumption at that time, which was shared by Gambaryan (1974) in his biomechanical considerations of a wide variety of mammals. More recent force-plate recordings, including those for pikas (Witte et al., 2002), show two significant patterns that challenge earlier assumptions. First, the vertical component of the ground reaction force in steady terrestrial walking and running is always several times greater than the horizontal component (longitudinal and transversal). Second, the longitudinal horizontal component is directed posteriorly (braking the body) in the first half of the contact phase, and anteriorly (accelerating the body) in the second half. This is true for both for the forelimb and the hindlimb. Therefore, the rule is not the deceleration by the forelimb and acceleration by the hindlimb, but deceleration in the first part of the contact phase of every limb and acceleration in the second part. It is also of note that large horizontal forces and accelerations take place not in steady running but at the rapid start and stop of the forward motion and in cornering. At the start and stop, longitudinal forces prevail, while in cornering the transverse forces are dominant. Thus, during cornering, the anterior part of the skull may tend to yaw centrifugally. In fact, Bramble (1989) has described the structure that prevents this distortion of the head, although he did not appreciate its adaptive role. In leporids, the os squamosum has a specific process, “the petrosal bar” which protrudes far posteriorly, crosses the piriform fenestra, and is housed by a matching “supratympanic sulcus” in the os petrosum. Therefore, the left and the right petrosal bars brace the basicranial block of the skull from both sides preventing lateral distortions in the kinetic zone in case of transverse accelerations. In such cases the heavy ears fall aside (Kuznetsov et al., 2017) and cannot help to save skull integrity.
Brain Evolution
Rabbits are well-known laboratory mammals for which there is an extensive historical record of the study of their brain morphology starting in the late 19th century (see Lewis, 1882; Mann, 1895; and references therein). Although the brain of the domesticated rabbit (O. cuniculus) is well studied, little is known about the evolution of the lagomorph brain. Until recently, data on brain morphology of fossil lagomorphs were derived from the fossil natural endocasts of Prolagus (Edinger, 1929), Hypolagus (Sych, 1967; Czyżewska, 1985), and Palaeolagus (Cope, 1884, but see Wood, 1940). These show similarities with modern lagomorph brains, such as narrow frontal lobes. The growing accessibility of μCT data has transformed the way we understand endocranial anatomy, especially regarding incorporation of quantitative data. The first virtual endocast of a fossil lagomorph, Megalagus turgidus, was described by López-Torres et al. (2020). The cranium of M. turgidus is the oldest (early Oligocene; Olson, 1942) and most complete lagomorph cranium for which mostly complete endocranial information can be recovered. Historically, M. turgidus has been classified as a leporid (Olson, 1942; Dawson, 1958, 2008), but more recently it has been considered to belong to a more primitive lineage outside crown lagomorphs (Lopez-Martinez, 2008). In the most comprehensive relevant phylogenetic analyses, the position of Megalagus as a stem lagomorph was supported (Fostowicz-Frelik, 2013; Fostowicz-Frelik and Meng, 2013). The study of the endocast of a stem lagomorph provides us with very valuable information on the brain morphology of lagomorphs before the split between the leporid and ochotonid lineages.
The endocast of Megalagus turgidus (Figure 5A) shows it had larger olfactory bulbs relative to its endocranial volume than any modern lagomorph. With respect to the cerebrum, the frontal lobes of Megalagus are wider than those observed in modern lagomorphs (narrow frontal lobes are a typical modern lagomorph trait), but they also are not as expanded rostrally, exposing much of the circular fissure. In contrast, ochotonids generally exhibit exposure of a portion of the circular fissure, and the circular fissure is covered in leporids (Figure 5B). The endocast of Megalagus possesses only a lateral sulcus on the neocortex, similar to the condition seen in leporids. The cerebral outline in dorsal view is markedly different among Megalagus, modern leporids, and modern ochotonids. The broadest point in the cerebrum of Megalagus is approximately midway between the rostral and caudal ends of the cerebrum, making the endocast have a rather ovoid outline. In contrast, leporids and ochotonids both have cerebra that are much broader in their caudal region (Figure 5). Therefore, there was likely a shift in lagomorph evolution to expand the caudal area of the cerebrum through time. In the caudal end of the cerebrum, the midbrain is partially exposed in Megalagus, similar to midbrain exposure in other primitive Euarchontoglires (e.g., most plesiadapiforms; Silcox et al., 2009, 2010; Orliac et al., 2014).
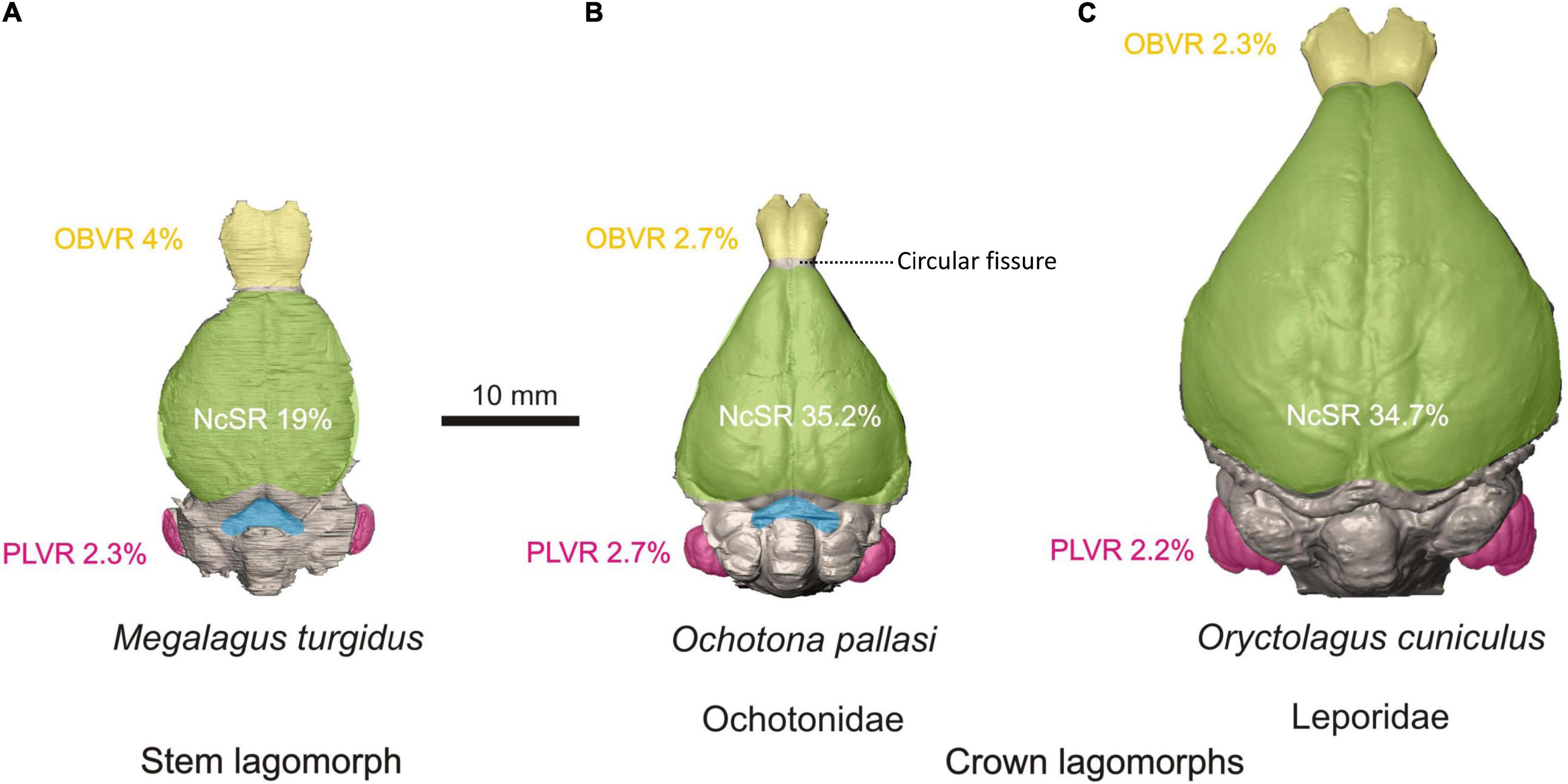
Figure 5. Comparative morphology of the brain endocast in stem lagomorphs. (A Megalagus turgidus) and crown clades: Ochotonidae (B Ochotona pallasi) and Leporidae (C Oryctolagus cuniculus). The colors mark the brain areas of particular interest: yellow, olfactory bulbs; green, neocortical surface; blue, midbrain exposure; pink, petrosal lobules. See the comparative metric data of selected indices: NcSR, neocortical surface ratio (the ratio of the neocortex surface area to total endocast surface area); OBVR, olfactory bulb volume ratio (the ratio of the olfactory bulb volume to the total endocast volume); PLVR, petrosal lobule volume ratio (the ratio of the petrosal lobule volume to the total endocast volume); all indices are percentage values. The images and metrical data are taken from López-Torres et al. (2020).
With respect to the modern lagomorph lineages, the range of relative olfactory bulb size of ochotonids is found within the range of leporids (López-Torres et al., 2020). In modern leporids, the midbrain is completely covered, and in modern ochotonids the midbrain is only slightly exposed (Figure 5C). The endocasts of ochotonids are completely lissencephalic, contrary to the endocasts of leporids, which only possess a lateral sulcus on the neocortex. The main difference in the cerebral outline between leporids and ochotonids is that the antero-lateral margins of the cerebrum in dorsal view are concave in leporids and either straight or slightly convex in ochotonids. This makes leporids have a rather pear-shaped dorsal outline, whereas in ochotonids it is more triangular (Figure 5). Modern lagomorph lineages also differ in that leporids have a proportionally longer (and larger) cerebrum than ochotonids (López-Torres et al., 2020).
Some tentative conclusions can be sketched about trends in the evolution of the lagomorph brain. Leporid and ochotonid lineages differ in many aspects of brain morphology, including the rostral expansion of the frontal lobes in leporids, a higher relative petrosal lobule volume in ochotonids, and the caudal expansion of the occipital region of the cerebrum in leporids. With respect to the latter, Kraatz et al. (2015) and Kraatz and Sherratt (2016) suggested a need for increased visual perception of the substrate in leporids, which might explain the more developed caudal region of the cerebrum in that group. In contrast, Megalagus exhibits a more ‘primitive’ brain, sharing more resemblances to endocasts that have been reconstructed for early fossil rodents (i.e., ischyromyids; Bertrand and Silcox, 2016; Bertrand et al., 2016, 2019) and plesiadapiforms (Silcox et al., 2009, 2010; Orliac et al., 2014). In particular, Megalagus has fairly large, pedunculated olfactory bulbs, some extent of midbrain exposure, and low neocorticalization. As in larger plesiadapiforms and rodents, Megalagus develops a lateral sulcus, with the brain being otherwise lissencephalic. These similarities with primitive members of Euarchontoglires, such as plesiadapiforms and primitive rodents, may indicate that lagomorphs are an old, relatively basal lineage and that they are conservative in their morphology.
Lagomorphs as Biomechanical Models
Rabbits were one of the first vertebrates for which the in vivo range of sarcomere shortening was precisely measured with diffraction techniques (Dimery, 1985). Contrary to the previous expectations, the shortening range in 12 hindlimb muscles appeared to be much less than 30%. The shortening employs the plateau of the well-known force-tension curve, which is the best for force production. Until now, similar data are available for very few other vertebrate species other than humans and rabbits (Burkholder and Lieber, 2001). Additionally, it has been shown that the number of successive sarcomeres in myofibrils directly depends on the excursions experienced by the muscle during an individual’s growth. Koh and Herzog (1998) released the musculus tibialis anterior from its retinacular restraint in 4-week-old rabbits. Free of the restraint, the muscle gained increased range for shortening and extension with the unchanged angular amplitude in the ankle joint. Twelve weeks later the operated muscles showed considerable difference from the control; muscle fibers became longer, while the tendons became shorter. As a byproduct, the force of operated muscle decreased. Rabbits have also proven to be a convenient model animal for human orthopedics. They are used in biomechanical modeling of the joints of both the spine (Grauer et al., 2000) and the limbs (Grover et al., 2007).
Cranial Biomechanics
Beyond those detailed by Bramble (1989), other morphological features of the cranium have been associated with locomotion including a pronounced ventral flexion of the facial region and unique fenestrations in the posterior cranial bones and lateral portion of the maxilla (DuBrul, 1950; Moss and Feliciano, 1977; Stott et al., 2010; Kraatz et al., 2015; Kraatz and Sherratt, 2016). The unique rostral vacuity (Ochotonidae) and fenestration (Leporidae) in lagomorphs were noted by Gray (1867), and DuBrul (1950) suggested that the latticing of the maxilla in Leporidae could increase the efficiency of speedy locomotion by reducing weight of the rostrum, and potentially contributing to Bramble’s (1989) anterior capital suspensory system hypothesis. However, Moss and Feliciano (1977) argued that these fenestrations are related to the lack of transmission of masticatory forces through the lateral aspect of the rostrum. They proposed that the more vertical orientation of the ramus of the mandible in some leporids redirects incisal forces along the dorsal and ventral aspects of the rostrum, and away from the lateral side. Although this has yet to be tested, methods such as in vivo strain-gage analysis coupled with in silico finite element analysis could provide more resolution.
Leporids process food through differing modes of mastication within a single bite cycle (Weijs, 1980), including incisor biting, molar crushing and molar shearing, and the potential influence of these modes on bone deposition and skull shape, including the fenestrated rostrum, remains unknown. Biomechanical modeling using multibody dynamics analysis has demonstrated varied muscle lines of action and bite force magnitudes and directions during a bite cycle (Watson et al., 2014). However, it is unknown whether the largest and/or more frequent masticatory strains across the whole cranium are generated through incisor biting, and how these are directed through the rostrum, or by crushing or shearing of food at the molars. What is evident is that the bony form of the circumorbital region, cranial vault and basicranium in lagomorphs is unlikely to be related to stresses experienced during biting and chewing (Jašarević et al., 2010; Franks et al., 2016, 2017). The debate about determinants of skull form, however, continues, as faster, saltatorial species such as hares and jackrabbits of the genus Lepus, have more extensive fenestration and a more pronounced ventral facial tilt (Kraatz et al., 2015; Kraatz and Sherratt, 2016). This is in comparison to generalists, including cottontail and pygmy rabbits (e.g., Sylvilagus spp. and B. idahoensis), suggesting that there may be a correlation between posture, locomotion, and rostral fenestrations as well as mastication.
Feeding Apparatus
In many ways, the function of the lagomorph oral cavity is especially well known among mammals. This is due largely to in vivo studies of jaw-adductor muscle activity, jaw kinematics and mandibular bone strain, and more recent work on diet-induced adaptive plasticity in domestic white rabbits (O. cuniculus). Rabbits exhibit a vertically deep facial skull and a jaw joint correspondingly elevated above the toothrow that that allows the jaw both rotational and translational movements (Weijs and Dantuma, 1981; Crompton et al., 2006). Transverse mandibular movements during the chewing cycle in rabbits are similar to primates in being due to delayed activity of the balancing-side deep-masseter muscle, which is in part related to dietary stiffness, i.e., elastic modulus (Weijs and Dantuma, 1981; Hylander et al., 1987, 2000; Weijs et al., 1989; Agrawal et al., 1997, 1998, 2000; Vinyard et al., 2006). Such kinematics are facilitated via a partially fused mandibular symphysis, a midline anteriorly located joint that closely binds the two jaw halves (Weijs and Dantuma, 1981; Ravosa and Hogue, 2004; Ravosa et al., 2016). Rabbits and diverse mammals have similar mandibular peak strains during the mastication of mechanically challenging foods (Weijs and de Jongh, 1977; Ravosa et al., 2010b). Rabbits share feeding patterns with other mammals whereby items with greater toughness and/or higher stiffness require larger and protracted jaw-adductor forces during biting and chewing, which results in greater occlusal forces and masticatory stresses (e.g., Herring and Scapino, 1973; Weijs and de Jongh, 1977; Hylander, 1979; Gorniak and Gans, 1980; Weijs and Dantuma, 1981; Dessem and Druzinsky, 1992; Hylander et al., 1992, 1998, 2000; Ravosa and Hogue, 2004; Vinyard et al., 2008). Rabbits are likewise noteworthy due to extensive ontogenetic data about the in vivo links among masticatory function, feeding behavior and food mechanical properties (e.g., Weijs et al., 1987, 1989; Langenbach et al., 1991, 2001; Langenbach and van Eijden, 2001; Martin et al., 2020).
Skeletal adaptations are related to either high-magnitude, singular loads or low-magnitude, cyclical loading. Although high bite forces have long been linked to greater jaw robusticity in mammals (e.g., Hylander, 1979), the dietary underpinnings of repetitive or cyclical loading are still poorly known for masticatory features. Rabbits are but one of two taxa for which mechanically challenging foods are known elevate cyclical loading (Ravosa et al., 2015; Nett et al., in press). Rabbits also resemble mid-to-large sized mammals in exhibiting intracortical remodeling, a skeletal repair mechanism related to microcrack damage in adult bone that does not appear to characterize smaller species (Bouvier and Hylander, 1996a,b; Hirano et al., 2000; Lad et al., 2021).
Rabbits are perhaps the best studied mammals in terms of dietary plasticity in the skull and jaws. A series of experiments have explored the implications of dynamic changes in elevated and cyclical loads on craniomandibular ontogeny and function in rabbits subjected to long-term, naturalistic modifications of dietary properties (Ravosa et al., 2007, 2016). Analyses have explored ontogenetic variation in jaw-loading patterns due to seasonality and fallback foods (Scott et al., 2014a, b); differences in age-related performance of bone and cartilage in jaw joints (Ravosa et al., 2007, 2008; Jašarević et al., 2010; Ravosa and Kane, 2017); reaction norms of bony vs. muscular components (Ravosa et al., 2010a); regional and hierarchical variation in patterns of cranial bone formation (Ravosa et al., 2007; Menegaz et al., 2009; Franks et al., 2016, 2017; Terhune et al., 2020; Lad et al., 2021); and morphological inference in the fossil record (Ravosa et al., 2016). Another finding is that dietary plasticity in rabbit jaws is similar to shorter-term studies of this phenomenon in monkeys, hyraxes, ferrets and rats (Beecher and Corruccini, 1981; Bouvier and Hylander, 1981, 1996a,b; Beecher et al., 1983; Yamada and Kimmel, 1991; He and Kiliaridis, 2003; Lieberman et al., 2004). These integrative analyses have marshaled a suite of histological, imaging and engineering approaches to understand masticatory development and phenotypic diversity in rabbits. Despite the differential focus of such research on the role of long-term plasticity in craniomandibular evolution and diversity, such an ontogenetic perspective to the mechanobiology of lagomorphs invariably lends itself to ongoing revolutions in ‘omics’ as well as clinical approaches to aging and pathobiology of connective tissues.
Clinical Model
Understanding of the healthy rabbit masticatory system and cranial morphology is important for diagnosis and treatment of dental disease in rabbits (Harcourt-Brown, 2007; Lennox, 2008; Jekl and Redrobe, 2013). The rabbit is also one of the most used animals in clinical experimental modeling (Neyt et al., 1998; Stübinger and Dard, 2013), often used to investigate the remodeling of bone in response to dental implants (Kim and Shin, 2018; Leventis et al., 2018; Manju et al., 2018) and impaired muscle function (Balanta-Melo et al., 2019; Huang et al., 2019). Rabbits are also a good clinical model for craniosynostosis, a condition that involves the premature closure of cranial sutures in infants. Craniosynostotic rabbits are the only existing colony of any species affected by familial craniosynostosis in the world (Mooney et al., 1998a, b; Cooper et al., 1999; Lyn Chong et al., 2003). Suture defects must be artificially created to investigate this question in any other species of mammal. Therefore, these rabbits are the most similar non-human model to naturally occurring craniosynostosis in other mammals and require less surgical manipulation.
Summary
Though model organisms are critical tools for understanding much of the biology of life, larger systems of organisms can offer a more dynamic and comparative framework to reveal the tempo and mode of evolutionary change. Understanding the adaptative processes that drive these changes is often best accomplished by studying groups of closely related organisms at multiple time scales. We offer the Lagomorpha (rabbits, hares, pikas, and their ancestors) as an exemplar system in which to study the evolutionary patterns of morphologic change. Anchored by the common laboratory rabbit (O. cuniculus), the order offers a rich diversity of extant species within the monogeneric Ochotonidae and the more lineage rich Leporidae. The molecular phylogenetics of these groups have been well studied, and indeed, four species have established genome level architecture. Understanding lagomorph intraordinal relationships has also advanced through a rich, consistent fossil record that dates back nearly 50 million years. The evolutionary trees are already in place to develop phylogenetically constrained models of evolution, but more work is needed combining molecular and morphological data into a comprehensive phylogeny of lagomorphs.
Using existing phylogenetic frameworks and emerging methods, lagomorphs are already an excellent system in which to study a rich array of functional systems. The locomotor behavior of these small mammals has been of long-standing interest, and research within this system has increasingly incorporated evidence from the fossil record, soft tissue systems, and novel connections to the cranium. The expanding research on the lagomorph cranium has shown important patterns of evolution of the brain and auditory systems and new methods have only increased the scope and breadth of research on the lagomorph masticatory system. The European rabbit will continue to remain a seminal research species for the biological sciences. Most importantly, new methods have allowed researchers to expand our knowledge more broadly among rabbit relatives, offering a more explicitly evolutionary context. The emergence of new methods of morphological research, an ever-improving phylogenetic framework, and a growing interest in lagomorphs will only serve to better place rabbits and all of their relatives as an essential system in which we can better understand the patterns and processes of evolutionary morphology.
Author Contributions
BK and IR designed the idea for the review manuscript. All authors contributed equally to the manuscript.
Funding
ES was supported by an Australian Research Council Future Fellowship (ARC FT190100803). RR was funded by the Alexander von Humboldt Foundation, which is sponsored by the Federal Ministry for Education and Research (Germany). Research discussed by MR herein was supported by the following United States National Science Foundation grants (BCS-1749453, BCS-1029149/1214767, and BCS-0924592/1214766).
Conflict of Interest
The authors declare that the research was conducted in the absence of any commercial or financial relationships that could be construed as a potential conflict of interest.
The reviewer TM declared a past co-authorship with one of the authors IR to the handling Editor.
Acknowledgments
We dedicate this paper to the late Mary R. Dawson (1931–2020) as a token of gratitude for her tremendous contribution to the morphological and evolutionary exploration on lagomorphs. We would like to thank the World Lagomorph Society and the organizers of the 6th World Lagomorph Conference, which was scheduled to occur in July of 2020 in Montpellier, France, but postponed due to the global pandemic of COVID-19. IR and BK had organized a session for that meeting titled Lagomorphs as a Model Morphological System. In lieu of that meeting and session, participants met virtually on July 06, 2020 to outline this review paper. We all look forward to gathering for a similar session in July of 2022 in Montpellier for the rescheduled World Lagomorph Conference. We thank reviewers for their thoughtful and helpful comments.
Footnote
References
Abbott, R., Albach, D., Ansell, S., Arntzen, J. W., Baird, S. J. E., Bierne, N., et al. (2013). Hybridization and speciation. J. Evol. Biol. 26, 229–246. doi: 10.1111/j.1420-9101.2012.02599.x
Agrawal, K. R., Lucas, P. W., and Bruce, I. C. (2000). The effects of food fragmentation index on mandibular closing angle in human mastication. Arch. Oral Biol. 45, 577–584. doi: 10.1016/S0003-9969(00)00019-4
Agrawal, K. R., Lucas, P. W., Bruce, I. C., and Prinz, J. F. (1998). Food properties that influence neuromuscular activity during human mastication. J. Dent. Res. 77, 1931–1938. doi: 10.1177/00220345980770111101
Agrawal, K. R., Lucas, P. W., Prinz, J. F., and Bruce, I. C. (1997). Mechanical properties of foods responsible for resisting food breakdown in the human mouth. Arch. Oral Biol. 42, 1–9. doi: 10.1016/S0003-9969(96)00102-1
Águeda-Pinto, A., Lemos, de Matos, A., Abrantes, M., Kraberger, S., Risalde, M. A., et al. (2019). Genetic characterization of a recombinant myxoma virus in the Iberian hare (Lepus granatensis). Viruses 11:530. doi: 10.3390/v11060530
Alliston, C. W., and Pardee, N. R. (1973). Variability of embryonic development in the rabbit at 19 to 168 hours after mating. Lab. Anim. Sci. 23, 665–670.
Alves, P. C., Ferrand, N., Suchentrunk, F., and Harris, D. J. (2003). Ancient introgression of Lepus timidus mtDNA into L. granatensis and L. europaeus in the Iberian Peninsula. Mol. Phylogenet. Evol. 27, 70–80. doi: 10.1016/S1055-7903(02)00417-7
Angermann, R., Flux, J. E. C., Chapman, J. A., and Smith, A. T. (1990). “Chapter 2: Lagomorph classification,” in Rabbits, Hares and Pikas. Status Survey and Conservation Action Plan, IUCN/SSC Lagomorph Specialist Group, eds J. A. Chapman and J. E. C. Flux (Oxford: Information Press), 7–13.
Asher, R. J., Meng, J., Wible, J. R., McKenna, M. C., Rougier, G. W., Dashzeveg, D., et al. (2005). Stem Lagomorpha and the antiquity of Glires. Science 307, 1091–1094. doi: 10.1126/science.1107808
Asher, R. J., Smith, M. R., Rankin, A., and Emry, R. J. (2019). Congruence, fossils and the evolutionary tree of rodents and lagomorphs. R. Soc. Open Sci. 6:190387. doi: 10.1098/rsos.190387
Averianov, A. O. (1994). Early eocene mimotonids of Kyrgyzstan and the problem of Mixodontia. Acta Palaeontol. Pol. 39, 393–411.
Averianov, A. O. (1995). Osteology and adaptations of the early Pliocene rabbit Trischizolagus dumitrescuae (Lagomorpha: Leporidae). J. Vertebr. Paleontol. 15, 375–386. doi: 10.1080/02724634.1995.10011236
Averianov, A. O., and Lopatin, A. V. (2005). Eocene Lagomorpha of Asia: 1. Aktashmys (Strenulagidae fam. nov.). Paleontol. J. 39, 308–317.
Averianov, A. O., and Tesakov, A. S. (1997). Evolutionary trends in Mio-Pliocene Leporinae, based on Trischizolagus (Mammalia, Lagomorpha). Paläontol. Z. 71, 145–153.
Balanta-Melo, J., Toro-Ibacache, V., Kupczik, K., and Buvinic, S. (2019). Mandibular bone loss after masticatory muscles intervention with botulinum toxin: an approach from basic research to clinical findings. Toxins 11:84. doi: 10.3390/toxins11020084
Barone, R., Pavaux, C., Blin, P. C., and Cuq, P. (1973). Atlas of Rabbit Anatomy. Paris: Masson et Cie.
Beecher, R. M., and Corruccini, R. S. (1981). Effects of dietary consistency on craniofacial and occlusal development in the rat. Angle Orthod. 51, 61–69. doi: 10.1043/0003-32191981051<0061:EODCOC<2.0.CO;2
Beecher, R. M., Corruccini, R. S., and Freeman, M. (1983). Craniofacial correlates of dietary consistency in a nonhuman primate. J. Cranl. Genet. Dev. Biol. 3, 193–202.
Bensley, B. A. (1921). Practical Anatomy of the Rabbit: An Elementary Laboratory Text Book in Mammalian Anatomy. Philadelphia: Blakiston Co.
Berkovitz, B., and Shellis, P. (2018). “Chapter 7 - Lagomorpha and Rodentia,” in The Teeth of Mammalian Vertebrates, eds B. Berkovitz and P. Shellis (Amsterdam: Elsevier Academic Press), 105–143.
Bertram, J. E., and Gutmann, A. (2009). Motions of the running horse and cheetah revisited: fundamental mechanics of the transverse and rotary gallop. J. R. Soc. Interface 6, 549–559. doi: 10.1098/rsif.2008.0328
Bertrand, O. C., Amador-Mughal, F., and Silcox, M. T. (2016). Virtual endocasts of Eocene Paramys (Paramyinae): oldest endocranial record for Rodentia and early brain evolution in Euarchontoglires. Proc. R. Soc. B 283:20152316. doi: 10.1098/rspb.2015.2316
Bertrand, O. C., Amador-Mughal, F., Lang, M. M., and Silcox, M. T. (2019). New virtual endocasts of Eocene Ischyromyidae and their relevance in evaluating neurological changes occurring through time in Rodentia. J. Mammal. Evol. 26, 345–371. doi: 10.1007/s10914-017-9425-6
Bertrand, O. C., and Silcox, M. T. (2016). First virtual endocasts of a fossil rodent: Ischyromys typus (Ischyromyidae, Oligocene) and brain evolution in rodents. J. Vertebr. Paleontol. 36:e1095762. doi: 10.1080/02724634.2016.1095762
Bleefeld, A. R., and Bock, W. J. (2002). Unique anatomy of lagomorph calcaneus. Acta Palaeontol. Pol. 47, 181–183.
Bohlin, B. (1942). The fossil mammals from the tertiary deposits of Taben-Buluk, western Kansu. Part 1. Insectivora and Lagomorpha. Palaeontol. Sin. C 8, 1–113.
Bohlin, B. (1951). “Some mammalian remains from Shih-ehr-ma-ch’eng, Hui-hui-p’u area, Western Kansu. Reports from the scientific expeditionto the North-Western Provinces of China under Leadership of Dr Sven Hedin,” in The Sino-Swedish Expedition Publication 35, VI. Vertebrate Paleontology 5, ed. S. Hedin (Stockholm: Hasse W. Tullberg), 1–48.
Böhmer, C., and Böhmer, E. (2017). Shape variation in the craniomandibular system and prevalence of dental problems in domestic rabbits: a case study in evolutionary veterinary science. Vet. Sci. 4:5. doi: 10.3390/vetsci4010005
Boussit, D. (1989). Reproduction et Insemination Artificielle en Cuniculture. Association Française de Cuniculture. France: Lempdes.
Bouvier, M., and Hylander, W. L. (1981). Effect of bone strain on cortical bone structure in macaques (Macaca mulatta). J. Morph. 167, 1–12.
Bouvier, M., and Hylander, W. L. (1996b). The mechanical or metabolic function of secondary osteonal bone in the monkey Macaca fascicularis. Arch. Oral Biol. 41, 941–950. doi: 10.1016/S0003-9969(96)00047-7
Bouvier, M., and Hylander, W. L. (1996a). “Strain gradients, age, and levels of modeling and remodeling in the facial bones of Macaca fascicularis,” in The Biological Mechanisms of Tooth Movement and Craniofacial Adaptation, eds Z. Davidovitch and L. A. Norton (Boston, MA: Harvard Society for the Advancement of Orthodontics), 407–412.
Bramble, D. M. (1989). Cranial specialization and locomotor habit in the Lagomorpha. Am. Zool. 29, 303–317. doi: 10.1093/icb/29.1.303
Bruce, N. W., and Abdul-Karim, R. W. (1973). Relationship between fetal weight, placental weight and maternal placental circulation in the rabbit at different stages of gestation. J. Reprod. Fertil. 32, 15–24. doi: 10.1530/jrf.0.0320015
Bugge, J. (1974). The cephalic arterial system in insectivores, primates, rodents and lagomorphs, with special reference to the systematic classification. Acta Anat. 87, 1–159.
Burgin, C. J., Colella, J. P., Kahn, P. L., and Upham, N. S. (2018). How many species of mammals are there? J. Mamm. 99, 1–14. doi: 10.1093/jmammal/gyx147
Burkholder, T. J., and Lieber, R. L. (2001). Sarcomere length operating range of vertebrate muscles during movement. J. Exp. Biol. 204, 1529–1536.
Camp, C. L., and Borell, A. E. (1937). Skeletal and muscular differences in the hind limbs of Lepus, Sylvilagus, and Ochotona. J. Mammal. 18, 315–326. doi: 10.2307/1374205
Carneiro, M., Albert, F. W., Afonso, S., Pereira, R. J., Burbano, H., Campos, R., et al. (2014). The genomic architecture of population divergence between subspecies of the European rabbit. PLoS Genet. 10:e1003519. doi: 10.1371/journal.pgen.1003519
Castillo Vardaro, J. A., Epps, C. W., Frable, B. W., and Ray, C. (2018). Identification of a contact zone and hybridization for two subspecies of the American pika (Ochotona princeps) within a single protected area. PLoS One 13:e0199032. doi: 10.1371/journal.pone.0199032
Cevegmid, D. (1950). Comparative Ecological-Morphological Analysis of the Organism of Pikas of Mongolia. Dissertation. Moscow: Moscow State University.
Chapman, J. A., and Ceballos, G. (1990). “The cottontails,” in Rabbits, Hares and Pikas, Status Survey and Conservation Action Plan: IUCN, eds J. A. Chapman and J. E. C. Flux (Gland: IUCN), 95–110.
Chapman, J. A., and Flux, J. E. C. (1990). “Introduction and overview of the lagomorphs,” in Rabbits, Hares and Pikas, Status Survey and Conservation Action Plan: IUCN, eds J. A. Chapman and J. E. C. Flux (Gland: IUCN), 1–6.
Chapman, J. A., and Morgan, R. P. (1973). Systematic status of the cottontail complex in western Maryland and nearby West Virginia. Wildlife Monogr. 36, 3–54.
Cheng, C., Ge, D. Y., Xia, L., Zhou, C. Q., and Yang, Q. S. (2012). Morphometrics study on the so called ‘Cape hare’ (Lagomorpha: Leporidae: Lepus) in China. Acta Theriol. Sin. 32, 275–286.
Climaco das Chagas, K. S., Vassallo, A. I., Becerra, F., Echeverría, A., de Castro Loguercio, M. F., et al. (2019). Locomotion in the fastest rodent, the mara Dolichotis patagonum (Caviomorpha; Caviidae; Dolichotinae). Mastozool. Neotrop. 26, 65–79.
Clutton-Brock, J. (1989). Five thousand years of livestock in Britain. Biol. J. Linn. Soc. 38, 31–37. doi: 10.1111/j.1095-8312.1989.tb01560.x
Cooper, G. M., Mooney, M. P., Burrows, A. M., Smith, T. D., Dechant, J., Losken, H. W., et al. (1999). Brain growth rates in craniosynostotic rabbits. Cleft Palate Craniofac. J. 36, 314–321. doi: 10.1597/1545-1569_1999_036_0314_bgricr_2.3.co_2
Cope, E. D. (1884). The Vertebrata of the tertiary formations of the West. Rep. United States Geol. Surv. Territ. 3, 1–35.
Corbet, G. B. (1983). A review of classification in the family Leporidae. Acta Zool. Fennica 174, 11–15.
Corbett-Detig, R. B., Russell, S. L., Nielsen, R., and Losos, J. (2020). Phenotypic convergence is not mirrored at the protein level in a lizard adaptive radiation. Mol. Biol. Evol. 37, 1604–1614. doi: 10.1093/molbev/msaa028
Crompton, A. W., Lieberman, D. E., and Aboelela, S. (2006). “Tooth orientation during occlusion and the functional significance of condylar translation in primates and herbivores,” in Amniote Paleobiology: Perspectives on the Evolution of Mammals, Birds, and Reptiles, eds M. T. Carrano, T. J. Gaudin, R. W. Blob, and J. R. Wible (Chicago, IL: University of Chicago Press), 367–388.
Czyżewska, T. (1985). Natural endocranial casts of Hypolagus brachygnathus Kormos, 1934 (Leporidae, Lagomorpha) from Wȩże I near Działoszyn. Acta Zool. Crac. 29, 1–12.
Dawson, M. R. (1958). Later Tertiary Leporidae of North America. Univ. Kansas Paleontol. Contr. Vertebr. 6, 1–75.
Dawson, M. R. (1969). Osteology of Prolagus sardus, a quaternary ochotonid (Mammalia, Lagomorpha). Palaeovertebrata 2, 157–191.
Dawson, M. R. (1981). “Evolution of modern leporids,” in Proceedings of the World Lagomorph Conference, eds K. Myers and C. D. MacInnes (Ontario: University of Guelph Press), 1–8.
Dawson, M. R. (2008). “Lagomorpha,” in Evolution of Tertiary Mammals of North America, Volume 2: Small Mammals, Xenarthrans, and Marine Mammals, eds C. M. Janis, G. F. Gunnell, and M. D. Uhen (Cambridge: Cambridge University Press), 293–310.
de Beer, G. R., and Woodger, J. H. (1930). The early development of the skull of the rabbit. Philos. Trans. R. Soc. Lond. B 218, 373–414. doi: 10.1098/rstb.1930.0009
de Jung, W. W., Gleaves, J. T., and Boulter, D. (1977). Evolutionary changes of alpha-crystallin and the phylogeny of mammalian orders. J. Mol. Evol. 10, 123–135. doi: 10.1007/BF01751806
Delaney, M. A., Treuting, P. M., and Rothenburger, J. L. (2018). “Chapter 19 – Lagomorpha,” in Pathology of Wildlife and Zoo Animals, eds K. A. Terio, D. McAloose, and J. S. Leger (Cambridge, MA: Academic Press), 481–496.
DeSesso, J. M. (1996). “Comparative embryology,” in Handbook of Developmental Toxicology, ed. R. D. Hood (Boca Raton, FL: CRC Press), 111–174.
Dessem, D., and Druzinsky, R. E. (1992). Jaw-muscle activity in ferrets, Mustela putorius furo. J. Morphol. 213, 275–286. doi: 10.1002/jmor.1052130211
Dimery, N. J. (1985). Muscle and sarcomere lengths in the hind limb of the rabbit (Oryctolagus cuniculus) during a galloping stride. J. Zool. 205, 373–383. doi: 10.1111/j.1469-7998.1985.tb05623.x
Douady, C. J., and Douzery, E. J. P. (2003). Molecular estimation of eulipotyphlan divergence times and the evolution of “Insectivora”. Mol. Phylogenet. Evol. 28, 285–296. doi: 10.1016/S1055-7903(03)00119-2
DuBrul, E. L. (1950). Posture, locomotion and the skull in Lagomorpha. Am. J. Anat. 87, 277–313. doi: 10.1002/aja.1000870205
Eloff, F. C. (1950). On the nasal region of the chondrocranium of the Cape hare, Lepus capensis. Ann. Transv. Mus. 21, 222–233.
Erbajeva, M. A. (2013). New species of Amphilagus (Lagomorpha, Mammalia) from the Miocene of the Valley of Lakes, central Mongolia. Paleontol. J. 47, 311–320. doi: 10.1134/S0031030113030040
Erbajeva, M. A., and Daxner-Höck, G. (2014). The most prominent Lagomorpha from the Oligocene and early Miocene of Mongolia. Ann. Naturhist. Mus. Wien 116, 215–245.
Erbajeva, M. A., Baatarjav, B., Daxner-Höck, G., and Flynn, L. J. (2017). Occurrences of Sinolagomys (Lagomorpha) from the Valley of Lakes (Mongolia). Palaeobiol. Palaeoenviron. 97, 11–24. doi: 10.1007/s12549-016-0262-z
Erbajeva, M., Angelone, C., and Alexeeva, N. (2016). A new species of the genus Amphilagus (Lagomorpha, Mammalia) from the Middle Miocene of south-eastern Siberia. Hist. Biol. 28, 199–207. doi: 10.1080/08912963.2015.1034119
Erbajeva, M., Flynn, L. J., and Alexeeva, N. (2015). Late cenozoic Asian ochotonidae: taxonomic diversity, chronological distribution and biostratigraphy. Quat. Int. 355, 18–23. doi: 10.1016/j.quaint.2014.10.064
Esselstyn, J. A., Oliveros, C. H., Swanson, M. T., and Faircloth, B. C. (2017). Investigating difficult nodes in the placental mammal tree with expanded taxon sampling and thousands of ultraconserved elements. Genome Biol. Evol. 9, 2308–2321. doi: 10.1093/gbe/evx168
Fa, J. E., and Bell, D. J. (1990). “The volcano rabbit Romerolagus diazi,” in Rabbits, Hares and Pikas, Status Survey and Conservation Action Plan: IUCN, eds J. A. Chapman and J. E. C. Flux (Gland: IUCN), 143–146.
Feijó, A., Ge, D., Wen, Z., Xia, L., and Yang, Q. (2020). Divergent adaptations in resource-use traits explain how pikas thrive on the roof of the world. Funct. Ecol. 34, 1826–1838. doi: 10.1111/1365-2435.13609
Fiorello, C. V., and German, R. Z. (1997). Heterochrony within species: craniofacial growth in giant, standard, and dwarf rabbits. Evolution 51, 250–261. doi: 10.1111/j.1558-5646.1997.tb02406.x
Flynn, L. J., Winkler, A. J., Erbaeva, M., Alexeeva, N., Anders, U., Cermák, S., et al. (2014). The Leporid Datum: a late Miocene biotic marker. Mammal Rev. 14, 164–176. doi: 10.1111/mam.12016
Foley, N. M., Springer, M. S., and Teeling, E. C. (2016). Mammal madness: is the mammal tree of life not yet resolved? Philos. Trans. R. Soc. Lond. B Biol. Sci. 371, 20150140. doi: 10.1098/rstb.2015.0140
Foote, R. H., and Carney, E. W. (2000). The rabbit as a model for reproductive and developmental toxicity studies. Reprod. Toxicol. 14, 477-493. doi: 10.1016/S0890-6238(00)00101-5
Forsyth, N. R., Elder, F. F. B., Shay, W. J., and Wright, E. W. (2005). Lagomorphs (rabbits, pikas and hares) do not use telomere-directed replicative aging in vitro. Mech. Ageing Dev. 126, 685–691. doi: 10.1016/j.mad.2005.01.003
Fostowicz-Frelik, Ł (2007). The hind limb skeleton and cursorial adaptations of the Plio–Pleistocene rabbit Hypolagus beremendensis. Acta Palaeontol. Pol. 52, 447–476.
Fostowicz-Frelik, Ł (2013). Reassessment of Chadrolagus and Litolagus (Mammalia: Lagomorpha) and a new genus of North American Eocene Lagomorpha from Wyoming. Am. Mus. 3773, 1–76. doi: 10.1206/3773.2
Fostowicz-Frelik, Ł (2016). A late Oligocene lagomorph (Mammalia) from Herrlingen 9 (Baden- Württemberg, Germany). Neues Jahrb. Geol. und Pal. Abh. 280, 143–151. doi: 10.1127/njgpa/2016/0571
Fostowicz-Frelik, Ł (2017). “Convergent and parallel evolution in early Glires (Mammalia),” in Evolutionary Biology: Self/Nonself Evolution, Species and Complex Traits Evolution, Methods and Concepts, ed. P. Pontarotti (Berlin: Springer International Publishing), 199–216.
Fostowicz-Frelik, Ł (2020). “Most successful mammals in the making: a review of the Paleocene Glires,” in Evolutionary Biology: A Transdisciplinary Approach, ed. P. Pontarotti (Cham: Springer Verlag Nature).
Fostowicz-Frelik, Ł, and Frelik, G. J. (2010). The earliest occurrence of the steppe pika (Ochotona pusilla) in Europe near the Pliocene/Pleistocene boundary. Naturwissenschaften 97, 325–329. doi: 10.1007/s00114-009-0634-6
Fostowicz-Frelik, Ł, and Li, Q. (2014). A new genus of stem lagomorph (Mammalia: Glires) from the Middle Eocene of the Erlian Basin, Nei Mongol, China. Acta Zool. Crac. 57, 29–42. doi: 10.3409/azc.57_1-2.29
Fostowicz-Frelik, Ł, and Meng, J. (2013). Comparative morphology of premolar foramen in lagomorphs (Mammalia: Glires) and its functional and phylogenetic implications. PLoS One 8:e79794. doi: 10.1371/journal.pone.0079794
Fostowicz-Frelik, Ł, Li, C. K., Li, Q., Meng, J., and Wang, Y. Q. (2015a). Strenulagus (Mammalia: Lagomorpha) from the middle Eocene Irdin Manha Formation of the Erlian Basin, Nei Mongol, China. Acta Geol. Sin. 89, 12–26. doi: 10.1111/1755-6724.12391
Fostowicz-Frelik, Ł, Li, C. K., Mao, F., Meng, J., and Wang, Y. Q. (2015b). A large mimotonid from the Middle Eocene of China sheds light on the evolution of lagomorphs and their kin. Sci. Rep. 5:9394. doi: 10.1038/srep09394
Fostowicz-Frelik, Ł, Li, C. K., Meng, J., and Wang, Y. Q. (2012). New Gobiolagus (Mammalia: Lagomorpha) material from the Middle Eocene of Erden Obo (Nei Mongol, China). Vertebr. PalAsiatica 50, 219–236.
Frahnert, S. (1998). Zur Stellung des Bibers (Castoridae: Castor) - Eine craniogenetische Studie zur Ethmoidalregion sciurognather Rodentia. Dissertation. Berlin: Humboldt-Universität Berlin.
Franks, E. M., Holton, N. E., Scott, J. E., McAbee, K. R., Rink, J. T., Pax, K., et al. (2016). Betwixt and between: intracranial perspective on zygomatic arch plasticity and function in mammals. Anat. Rec. 299, 1646–1660. doi: 10.1002/ar.23477
Franks, E. M., Scott, J. E., McAbee, K. R., Scollan, J. P., Eastman, M. M., and Ravosa, M. J. (2017). Intracranial and hierarchical perspective on dietary plasticity in mammals. Zoology 124, 30–41. doi: 10.1016/j.zool.2017.03.003
Frick, H., and Heckmann, U. (1955). Ein beitrag zur morphogenese des kaninchenschädels. Acta Anat. 24, 268–314. doi: 10.1159/000141047
Garland, T. (1983). The relation between maximal running speed and body mass in terrestrial mammals. J. Zool. 199, 157–170. doi: 10.1111/j.1469-7998.1983.tb02087.x
Gaupp, E. (1906). “Die Entwickelung des Kopfkelettes,” in Handbuch der vergleichenden und experimentellen Entwickelungslehre der Wirbeltiere, Vol. 3, ed. O. Hertwig (Jena: Fischer), 573–874.
Gawne, C. E. (1978). Leporids (Lagomorpha, Mammalia) from the Chadronian (Oligocene) deposits of Flagstaff Rim, Wyoming. J. Paleontol. 52, 1103–1118.
Ge, D. Y., Lissovsky, A. A., Xia, L., Cheng, C., and Smith, A. T. (2012). Reevaluation of several taxa of Chinese lagomorphs (Mammalia: Lagomorpha) described on the basis of pelage phenotype variation. Mammal Biol. 77, 113–123. doi: 10.1016/j.mambio.2011.09.009
Ge, D. Y., Wen, Z. X., Xia, L., Zhang, Z. Q., Erbajeva, M., Hung, C. M., et al. (2013). Evolutionary history of lagomorphs in response to global environmental change. PLoS One 8:e59668. doi: 10.1371/journal.pone.0059668
Ge, D. Y., Yao, L., Xia, L., Zhang, Z. Q., and Yang, Q. S. (2015). Geometric morphometric analysis of skull morphology reveals loss of phylogenetic signal at the generic level in extant lagomorphs (Mammalia: Lagomorpha). Contr. Zool. 84, 267–284. doi: 10.1163/18759866-08404001
Genereux, D. P., Serres, A., Armstrong, J., Johnson, J., Marinescu, V. D., Murén, E., et al. (2020). A comparative genomics multitool for scientific discovery and conservation. Nature 587, 240–245. doi: 10.1038/s41586-020-2876-6
Gignac, P. M., Kley, N. J., Clarke, J. A., Colbert, M. W., Morhardt, A. C., Cerio, D., et al. (2016). Diffusible iodine-based contrast-enhanced computed tomography (diceCT): an emerging tool for rapid, high-resolution, 3-D imaging of metazoan soft tissues. J. Anat. 228, 889–909. doi: 10.1111/joa.12449
Giska, I., Farelo, L., Pimenta, J., Seixas, F. A., Ferreira, M. S., Marques, J. P., et al. (2019). Introgression drives repeated evolution of winter coat color polymorphism in hares. PNAS 116, 24150–24156. doi: 10.1073/pnas.1910471116
Gorniak, G. C., and Gans, C. (1980). Quantitative assay of electromyograms during mastication in domestic cats (Felis catus). J. Morphol. 163, 253–281. doi: 10.1002/jmor.1051630304
Graham, J. E. (2015). “Chapter 41 - Lagomorpha (Pikas, Rabbits, and Hares),” in Fowler’s Zoo and Wild Animal Medicine, Vol. 8, eds R. E. Miller and M. E. Fowler (Amsterdam: Elsevier), 8375–8384.
Grauer, J. N., Erulkar, J. S., Patel, T. C., and Panjabi, M. M. (2000). Biomechanical evaluation of the New Zealand white rabbit lumbar spine: a physiologic characterization. Eur. Spine J. 9, 250–255. doi: 10.1007/s005860000141
Graur, D., Duret, L., and Gouy, M. (1996). Phylogenetic position of the order Lagomorpha (rabbits, hares and allies). Nature 379, 333–335. doi: 10.1038/379333a0
Gray, J. E. (1867). XXVII.—Notes on the skulls of hares (Leporidæ) and picas (Lagomyidæ) in the British Museum. Ann. Magaz. Nat. Hist. 20, 219–225. doi: 10.1080/00222936708694118
Grover, D. M., Chen, A. A., and Hazelwood, S. J. (2007). Biomechanics of the rabbit knee and ankle: muscle, ligament, and joint contact force predictions. J. Biomech. 40, 2816–2821. doi: 10.1016/j.jbiomech.2007.01.002
Hackert, R., Witte, H., and Fischer, M. S. (2006). “Interactions between motions of the trunk and the angle of attack of the forelimbs in synchronous gaits of the Pika (Ochotona rufescens),” in Adaptive Motion of Animals and Machines, eds H. Kimura, K. Tsuchiya, A. Ishiguro, and A. H. Witt (Tokyo: Springer), 69–77.
Halanych, K., Demboski, J., Van Vuuren, B., Klein, D., and Cook, J. (1999). Cytochrome b phylogeny of North American hares and jackrabbits (Lepus, Lagomorpha) and the effects of saturation in outgroup taxa. Mol. Phylogenet. Evol. 11, 213–221. doi: 10.1006/mpev.1998.0581
Hall, B. K. (2005). Bones and Cartilage: Developmental and Evolutionary Skeletal Biology. Amsterdam: Academic Press.
Harcourt-Brown, F. M. (2007). The progressive syndrome of acquired dental disease in rabbits. J. Exot. Pet. Med. 16, 146–157. doi: 10.1053/j.jepm.2007.06.003
Harper, M. J. (1961). The time of ovulation in the rabbit following the injection of luteinizing hormone. J. Endocrinol. 22, 147-152. doi: 10.1677/joe.0.0220147
He, T., and Kiliaridis, S. (2003). Effects of masticatory muscle function on craniofacial morphology on growing ferrets (Mustela putorius furo). Eur. J. Oral Sci. 11, 510–517. doi: 10.1111/j.0909-8836.2003.00080.x
Hecker, N., Sharma, V., and Hiller, M. (2019). Convergent gene losses illuminate metabolic and physiological changes in herbivores and carnivores. PNAS 116, 3036–3041. doi: 10.1073/pnas.1818504116
Heissig, K., and Schmidt-Kittler, N. (1975). Ein primitiver Lagomorphe aus dem Mitteloligozän Süddeutschlands. Mitt. Bayer. Staatsml. Pal. Hist. Geol. 15, 57–62.
Heissig, K., and Schmidt-Kittler, N. (1976). Neue Lagomorphen-funde aus dem Mitteloligozän. Mitt. Bayer. Staatsml. Pal. Hist. Geol. 16, 83–93.
Herring, S. W., and Scapino, R. P. (1973). Physiology of feeding in miniature pigs. J. Morphol. 141, 427–460. doi: 10.1002/jmor.1051410405
Hibbard, C. W. (1963). The origin of the p3 pattern of Sylvilagus, Caprolagus, Oryctolagus and Lepus. J. Mammal. 44, 1–15. doi: 10.2307/1377162
Hirano, T., Burr, D. B., Cain, R. L., and Hock, J. M. (2000). Changes in geometry and cortical porosity in adult, ovary-intact rabbits after 5 months treatment with LY333334 (hPTH 1-34). Calcif. Tissue Int. 66, 456–460. doi: 10.1007/s002230010091
Hoffmann, R. S., and Smith, A. T. (2005). “Order Lagomorpha,” in The Mammal Species of the World: A Taxonomic and Geographic Reference, eds D. E. Wilson and D. M. Reeder (London: Johns Hopkins, University Press), 185–211.
Hoyte, D. A. N. (1961). The postnatal growth of the ear capsule in the rabbit. Am. J. Anat. 108, 1–16. doi: 10.1002/aja.1001080102
Huang, D., Wu, Q., Zhou, X., and Kang, F. (2019). Activity and morphologic changes in the mandible after mandibular osteotomy. Am. J. Orthodont. Dentofac. Orthoped. 155, 40–47. doi: 10.1016/j.ajodo.2018.02.016
Huang, X. S. (1987). Fossil ochotonids from the middle Oligocene of Ulantatal, Nei Mongol. Vert. PalAsiatica 25, 260–282.
Huchon, D., Madsen, O., Sibbald, M. J. J. B., Ament, K., Stanhope, M. J., Catzeflis, F., et al. (2002). Rodent phylogeny and a timescale for the evolution of Glires: evidence from an extensive taxon sampling using three nuclear genes. Mol. Biol. Evol. 19, 1053–1065. doi: 10.1093/oxfordjournals.molbev.a004164
Hylander, W. L. (1979). The functional significance of primate mandibular form. J. Morphol. 160, 223–240. doi: 10.1002/jmor.1051600208
Hylander, W. L., Johnson, K. R., and Crompton, A. W. (1987). Loading patterns and jaw movements during mastication in Macaca fascicularis: a bone-strain, electromyographic and cineradiographic analysis. Am. J. Phys. Anthropol. 72, 287–314. doi: 10.1002/ajpa.1330720304
Hylander, W. L., Johnson, K. R., and Crompton, A. W. (1992). Muscle force recruitment and biomechanical modeling: an analysis of masseter muscle function during mastication in Macaca fascicularis. Am. J. Phys. Anthropol. 88, 365–387. doi: 10.1002/ajpa.1330880309
Hylander, W. L., Ravosa, M. J., Ross, C. F., and Johnson, K. R. (1998). Mandibular corpus strain in primates: further evidence for a functional link between symphyseal fusion and jaw-adductor muscle force. Am. J. Phys. Anthropol. 107, 257–271. doi: 10.1002/(SICI)1096-8644(199811)107:3<257::AID-AJPA3<3.0.CO;2-6
Hylander, W. L., Ravosa, M. J., Ross, C. F., Wall, C. E., and Johnson, K. R. (2000). Symphyseal fusion and jaw-adductor muscle force: an EMG study. Am. J. Phys. Anthropol. 112, 469–492. doi: 10.1002/1096-8644(200008)112:4<469::AID-AJPA5<3.0.CO;2-V
Insom, E., Magnoni, M. L., and Simonetta, A. M. (1990). Etudes sur la morphologie évolutive des Ochotonidés (Mammalia, Lagomorpha). 1. La morphologie crânienne d’Ochotona rufescens et d’Ochotona roylei. Mammalia 54, 633–651. doi: 10.1515/mamm.1990.54.4.633
Jašarević, E., Ning, J., Daniel, A. N., Menegaz, R. A., Johnson, J. J., Stack, M. S., et al. (2010). Masticatory loading, function and plasticity: a microanatomical analysis of mammalian circumorbital soft-tissue structures. Anat. Rec. 293, 642–650. doi: 10.1002/ar.21135
Jekl, V., and Redrobe, S. (2013). Rabbit dental disease and calcium metabolism - the science behind divided opinions. J. Small Anim. Pract. 54, 481–490. doi: 10.1111/jsap.12124
Jones, M. R., Mills, L. S., Alves, P. C., Callahan, C. M., Alves, J. M., Lafferty, D. J. R., et al. (2018). Adaptive introgression underlies polymorphic seasonal camouflage in snowshoe hares. Science 360, 1355–1358. doi: 10.1126/science.aar5273
Jones, M. R., Mills, L. S., Jensen, J. D., and Good, J. M. (2020). Convergent evolution of seasonal camouflage in response to reduced snow cover across the snowshoe hare range. Evolution 74, 2033–2045. doi: 10.1111/evo.13976
Kennelly, J. J., Foote, R. H., and Jones, R. C. (1970). Duration of premeiotic deoxyribonucleic acid synthesis and the stages of prophase I in rabbit oocytes. J. Cell. Biol. 47, 577–584. doi: 10.1083/jcb.47.3.577
Kim, I.-J., and Shin, S.-Y. (2018). Comparative study of new bone formation capability of zirconia bone graft material in rabbit calvarial. J. Adv. Prosthodont. 10, 167–176. doi: 10.4047/jap.2018.10.3.167
Kinoshita, G., Nunome, M., Kryukov, A. P., Kartavtseva, I. V., Han, S. H., Yamada, F., et al. (2019). Contrasting phylogeographic histories between the continent and islands of East Asia: massive mitochondrial introgression and long-term isolation of hares (Lagomorpha: Lepus). Mol. Phylogenet. Evol. 136, 65–75. doi: 10.1016/j.ympev.2019.04.003
Klebanova, E. A., Polyakova, R. S., and Sokolov, A. S. (1971). Morpho-functional features of the organs of support and locomotion in lagomorphs. Trans. Zool. Inst. 48, 58–120.
Koenigswald, W. V., Anders, U., Engels, S., Schultz, J. A., and Ruf, I. (2010). Tooth morphology in fossil and extant Lagomorpha (Mammalia) reflects different mastication patterns. J. Mammal. Evol. 17, 275–299. doi: 10.1007/s10914-010-9140-z
Koh, T. J., and Herzog, W. (1998). Excursion is important in regulating sarcomere number in the growing rabbit tibialis anterior. J. Physiol. 508, 267–280. doi: 10.1111/j.1469-7793.1998.267br.x
Koju, N. P., He, K., Chalise, M. K., Ray, C., Chen, Z., Zhang, B., et al. (2017). Multilocus approaches reveal underestimated species diversity and inter-specific gene flow in pikas (Ochotona) from southwestern China. Mol. Phylogenet. Evol. 107, 239–245. doi: 10.1016/j.ympev.2016.11.005
Kraatz, B. P., and Sherratt, E. (2016). Evolutionary morphology of the rabbit skull. PeerJ 4:e2453. doi: 10.7717/peerj.2453
Kraatz, B. P., Meng, J., Weksler, M., and Li, C. (2010). Evolutionary patterns in the dentition of Duplicidentata (Mammalia) and a novel trend in the molarization of premolars. PLoS One 5:e12838. doi: 10.1371/journal.pone.0012838
Kraatz, B. P., Sherratt, E., Bumacod, N., and Wedel, M. J. (2015). Ecological correlates to cranial morphology in leporids (Mammalia, Lagomorpha). PeerJ 3:e844. doi: 10.7717/peerj.844
Kriegs, J. O., Zemann, A., Churakov, G., Matzke, A., Ohme, M., Zischler, H., et al. (2010). Retroposon insertions provide insights into deep lagomorph evolution. Mol. Biol. Evol. 27, 2678–2681. doi: 10.1093/molbev/msq162
Kullberg, M., Nilsson, M. A., Arnason, U., Harley, E. H., and Janke, A. (2006). Housekeeping genes for phylogenetic analysis of eutherian relationships. Mol. Biol. Evol. 23, 1493–1503. doi: 10.1093/molbev/msl027
Kumar, V., Hallstrom, B. M., and Janke, A. (2013). Coalescent-based genome analyses resolve the early branches of the Euarchontoglires. PLoS One 8:e60019. doi: 10.1371/journal.pone.0060019
Kuznetsov, A. N., Luchkina, O. S., Panyutina, A. A., and Kryukova, N. V. (2017). Observations on escape runs in wild European hare as a basis for the mechanical concept of extreme cornering with special inference of a role of the peculiar subclavian muscle. Mamm. Biol. 84, 61–72. doi: 10.1016/j.mambio.2017.01.003
Lad, S. E., Anderson, R. J., Cortese, S. A., Alvarez, C. E., Danison, A. D., Morris, H. M., et al. (2021). Bone remodeling and cyclical loading in maxillae of New Zealand white rabbits (Oryctolagus cuniculus). Anat. Rec. [Epub ahead of print] doi: 10.1002/ar.24599
Lado, S., Alves, P. C., Brito, J., et al. (2016). Population history and taxonomy of African hares (genus Lepus) inferred from genetic variation. Mamm. Biol. 81:12. doi: 10.1016/j.mambio.2016.07.035
Lammers, A. R., and German, R. Z. (2002). Ontogenetic allometry in the locomotor skeleton of specialized half-bounding mammals. J. Zool. 258, 485–495. doi: 10.1017/S0952836902001644
Langenbach, G. E. J., and van Eijden, T. M. G. J. (2001). Mammalian feeding motor patterns. Am. Zool. 41, 1338–1351. doi: 10.1093/icb/41.6.1338
Langenbach, G. E. J., Weijs, W. A., and Koolstra, J. H. (1991). Biomechanical changes in the rabbit masticatory system during postnatal development. Anat. Rec. 230, 406–416. doi: 10.1002/ar.1092300313
Langenbach, G. E. J., Weijs, W. A., Brugman, P., and van Eijden, T. M. G. J. (2001). A longitudinal electromyographic study of the postnatal maturation of mastication in the rabbit. Arch. Oral Biol. 46, 11–20. doi: 10.1016/S0003-9969(01)00043-7
Lanier, H. C., and Olson, L. E. (2009). Inferring divergence times within pikas (Ochotona spp.) using mtDNA and relaxed molecular dating techniques. Mol. Phylogenet. Evol. 53, 1–12. doi: 10.1016/j.ympev.2009.05.035
Lanzetti, A., Berta, A., and Ekdale, E. G. (2018). Prenatal development of the humpback whale: growth rate, tooth loss, and skull shape changes in an evolutionary framework. Anat. Rec. 303, 180–204. doi: 10.1002/ar.23990
Lennox, A. M. (2008). Diagnosis and treatment of dental disease in pet rabbits. J. Exot. Pet. Med. 17, 107–113. doi: 10.1053/j.jepm.2008.03.008
Leonelli, S., and Ankeny, R. A. (2013). What makes a model organism? Endeavour 37, 209–212. doi: 10.1016/j.endeavour.2013.06.001
Levanen, R., Thulin, C. G., Spong, G., and Pohjoismaki, J. L. O. (2018). Widespread introgression of mountain hare genes into Fennoscandian brown hare populations. PLoS One 13:e0191790. doi: 10.1371/journal.pone.0191790
Leventis, M., Fairbairn, P., Mangham, C., Galanos, A., Vasiliadis, O., Papavasileiou, D., et al. (2018). Bone healing in rabbit calvaria defects using a synthetic bone substitute: a histological and micro-CT comparative study. Materials 11:2004. doi: 10.3390/ma11102004
Lewis, W. B. (1882). On the comparative structure of the brain in rodents. Philos. Trans. R. Soc. Lond. 173, 699–746. doi: 10.1098/rspl.1881.0066
Li, C. K. (1977). Paleocene eurymyloids (Anagalida, Mammalia) of Qianshan, Anhui. Vertebr. PalAsiatica 15, 103–118.
Li, C. K., and Ting, S. Y. (1985). “Possible phylogenetic relationships of eurymylids and rodents, with comments on mimotonids,” in Evolutionary Relationships Among Rodents: A Multidisciplinary Analysis, eds W. P. Luckett and J. L. Hartenberger (New York, NY: Plenum Press), 35–58.
Li, C. K., Meng, J., and Wang, Y. Q. (2007). Dawsonolagus antiquus, a primitive lagomorph from the eocenetheeocene arshanto formation, Nei Mongol, China. Bull. Carnegie Mus. 39, 97–110. doi: 10.2992/0145-9058200739[97:DAAPLF]2.0.CO;2
Li, C. K., Wang, Y. Q., Zhang, Z. Q., Mao, F. Y., and Meng, J. (2016). A new mimotonidan mammal Mina hui (Mammalia, Glires) from the Middle Paleocene of Qianshan, Anhui Province, China. Vertebr. PalAsiatica 54, 121–136.
Li, Q., Wang, Y. Q., and Fostowicz-Frelik, Ł (2016). Small mammal fauna from Wulanhuxiu (Nei Mongol, China) implies the Irdinmanhan–Sharamurunian (Eocene) faunal turnover. Acta Palaeontol. Pol. 61, 759–776. doi: 10.4202/app.00292.2016
Lieberman, D. E., Krovitz, G. E., Yates, F., Devlin, M., and St Claire, M. (2004). Effects of food processing on masticatory strain and craniofacial growth in a retrognathic face. J. Hum. Evol. 46, 655–677. doi: 10.1016/j.jhevol.2004.03.005
Lin, Y.-H., Waddell, P. J., and Penny, D. (2002). Pika and vole mitochondrial genomes increase support for both rodent monophyly and Glires. Gene 294, 119–129. doi: 10.1016/S0378-1119(02)00695-9
Lissovsky, A. (2014). Taxonomic revision of pikas Ochotona (Lagomorpha, Mammalia) at the species level. Mammalia 78, 199–216. doi: 10.1515/mammalia-2012-0134
Lissovsky, A. A. (2016). “Ochotonidae (pikas),” in Handbook of the Mammals of the World. Lagomorphs and Rodents I, Vol. 6, eds D. E. Wilson, T. E. Lacher, and R. A. Mittermeier (Barcelona: Lynx Edicions), 28–60.
Lissovsky, A. A., Yatsentyuk, S. P., and Koju, N. P. (2019). Multilocus phylogeny and taxonomy of pikas of the subgenus Ochotona (Lagomorpha. Ochotonidae). Zool. Scr. 48, 1–16. doi: 10.1111/zsc.12325
Liu, J., Yu, L., Arnold, M., Wu, C.-H., Wu, S.-F., Lu, X., et al. (2011). Reticulate evolution: frequent introgressive hybridization among chinese hares (genus Lepus) revealed by analyses of multiple mitochondrial and nuclear DNA loci. BMC Evol. Biol. 11:223. doi: 10.1186/1471-2148-11-223
Lopatin, A. V. (1998). A revision of the early Miocene Lagomorpha (Mammalia) from the North Aral region. Paleont. J. C 32, 291–304.
Lopatin, A. V., and Averianov, A. O. (2008). The earliest lagomorph (Lagomorpha, Mammalia) from the basal Eocene of Mongolia. Doklady Biol. Sci. 419, 131–132.
Lopatin, A., and Averianov, A. O. (2020). Arnebolagus, the oldest eulagomorph, and phylogenetic relationships within the Eocene Eulagomorpha new clade (Mammalia, Duplicidentata). J. Paleontol. 95, 394–405. doi: 10.1017/jpa.2020.94
López Martínez, N. (1985). “Reconstruction of the ancestral cranioskeletal features in the order Lagomorpha,” in Evolutionary Relationships Among Rodents: A Multidisciplinary Analysis, eds W. P. Luckett and J.-L. Hartenberger (New York, NY: Plenum Press), 151–189.
López Martínez, N. (2001). Paleobiogeographical history of Prolagus, a European ochotonid (Lagomorpha). Lynx 32, 215–231.
Lopez-Bejar, M. (1995). Evaluacion de la Viabilidad de Embriones de Conejo de Diversos Estadios Preimplantaciones Crioconservados Mediante Procedimientos de la Congelacion Rapida. Disseration. Barcelona: Universidad Autònoma de Barcelona.
Lopez-Martinez, N. (2008). “The lagomorph fossil record and the origin of the European rabbit,” in Lagomorph Biology: Evolution, Ecology, and Conservation, eds P. C. Alves, N. Ferrand, and K. Hackländer (Berlin: Springer-Verlag), 27–46.
López-Torres, S., Bertrand, O. C., Lang, M. M., Silcox, M. T., and Fostowicz-Frelik, Ł (2020). Cranial endocast of the stem lagomorph Megalagus and brain structure of basal Euarchontoglires. Proc. R. Soc. B 287:20200665. doi: 10.1098/rspb.2020.0665
Losos, J. B. (2011). Lizards in an Evolutionary Tree: Ecology and Adaptive Radiation of Anoles. Berkeley: University of California Press.
Lu, X. (2003). Postnatal growth of skull linear measurements of Cape Hare Lepus capensis in northern China: an analysis in adaptive context. Biol. J. Linn. Soc. 78, 343–353. doi: 10.1046/j.1095-8312.2003.00145.x
Lyn Chong, S., Mitchell, R., Moursi, A. M., Winnard, P., Losken, H. W., Bradley, J., et al. (2003). Rescue of coronal suture fusion using transforming growth factor-beta 3 (Tgf-β3) in rabbits with delayed-onset craniosynostosis. Anat. Rec. A 274A, 962–971. doi: 10.1002/ar.a.10113
Magne de la Croix, P. (1928). Sobre la evolución del galope de carrera y la consecutiva de la forma causa de la evolución en perisodáctiles y artiodáctiles. Anal. Soc. Cient. Argentina 106, 317–331.
Magne de la Croix, P. (1933). Des retours de l’évolution et la relativité des théories. Anal. Soc. Cient. Argentina 116, 225–239.
Magne de la Croix, P. (1936). The evolution of locomotion in mammals. J. Mammal. 17, 51–54. doi: 10.2307/1374551
Maier, W., and Ruf, I. (2014). Morphology of the nasal capsule of Primates - with special reference to Daubentonia and Homo. Anat. Rec. 297, 1985–2006. doi: 10.1002/ar.23023
Maier, W., Klingler, P., and Ruf, I. (2002). Ontogeny of the medial masseter muscle, pseudo-myomorphy, and the systematic position of the Gliridae (Rodentia, Mammalia). J. Mamm. Evol. 9, 253–269. doi: 10.1023/A:1023979212759
Maier, W., Tröscher, A., and Ruf, I. (2018). The anterior process of the malleus in extant Lagomorpha (Mammalia). J. Morphol. 279, 132–146. doi: 10.1002/jmor.20759
Manju, V., Anitha, A., Menon, D., Iyer, S., Nair, S. V., and Nair, M. B. (2018). Nanofibrous yarn reinforced HA-gelatin composite scaffolds promote bone formation in critical sized alveolar defects in rabbit model. Biomed. Mater. 13:065011. doi: 10.1088/1748-605X/aadf99
Mann, G. (1895). Homoplasy of the brain of rodents, insectivores, and carnivores. J. Anat. Physiol. 30, 1–36.
Marques, J. P., Farelo, L., Vilela, J., Vanderpool, D., Alves, P. C., Good, J. M., et al. (2017). Range expansion underlies historical introgressive hybridization in the Iberian hare. Sci. Rep. 7:40788. doi: 10.1038/srep40788
Marques, J. P., Seixas, F. A., Farelo, L., Callahan, C. M., Good, J. M., Montgomery, W. I., et al. (2020). An annotated draft genome of the mountain hare (Lepus timidus). Genome Biol. Evol. 12, 3656–3662. doi: 10.1093/gbe/evz273
Martin, L. F., Krause, L., Ulbricht, A., Winkler, D. E., Codrone, D., Kaiser, T. M., et al. (2020). Dental wear at macro- and micoscopic scale in rabbits fed diets of different abrasiveness: a pilot investigation. Palaeogeogr. Palaeocl. 556:109886. doi: 10.1016/j.palaeo.2020.109886
Martin, T. (1999). Phylogenetic implications of Glires (Eurymylidae, Mimotonidae, Rodentia, Lagomorpha) incisor enamel microstructure. Zoosyst. Evol. 75, 257–273. doi: 10.1002/mmnz.19990750207
Martin, T. (2004). Evolution of incisor enamel microstructure in Lagomorpha. J. Vertebr. Paleontol. 24, 411–426.
Matthee, C. A., Van Vuuren, B. J., Bell, D., and Robinson, T. J. (2004). A molecular supermatrix of the rabbits and hares (Leporidae) allows for the identification of five intercontinental exchanges during the Miocene. Syst. Biol. 53, 433–447. doi: 10.1080/10635150490445715
Mauleon, P. (1967). Différenciation et évolution des cellules sexuelles. La lignée femelle. Arch. Anat. Microsc. 56, 125–150.
McKenna, M. C. (1982). Lagomorph interrelationships. Geobios 15, 213–223. doi: 10.1016/S0016-6995(82)80115-0
Melo-Ferreira, J., Boursot, P., Carneiro, M., Esteves, P. J., Farelo, L., and Alves, P. C. (2012). Recurrent introgression of mitochondrial DNA among hares (Lepus spp.) revealed by species-tree inference and coalescent simulations. Syst. Biol. 61, 367–381. doi: 10.1093/sysbio/syr114
Melo-Ferreira, J., Farelo, L., Freitas, H., Suchentrunk, F., Boursot, P., and Alves, P. C. (2014a). Home-loving boreal hare mitochondria survived several invasions in Iberia: the relative roles of recurrent hybridisation and allele surfing. Heredity 112, 265–273. doi: 10.1038/hdy.2013.102
Melo-Ferreira, J., Seixas, F. A., Cheng, E., Mills, L. S., and Alves, P. C. (2014b). The hidden history of the snowshoe hare, Lepus americanus: extensive mitochondrial DNA introgression inferred from multilocus genetic variation. Mol. Ecol. 23, 4617–4630. doi: 10.1111/mec.12886
Menegaz, R. A., Sublett, S. V., Figueroa, S. D., Hoffman, T. J., and Ravosa, M. J. (2009). Phenotypic plasticity and function of the hard palate in growing rabbits. Anat. Rec. 292, 277–284. doi: 10.1002/ar.20840
Meng, J., and Wyss, A. R. (2001). The morpology of Tribosphenomys (Rodentiaformes, Mammalia): phylogenetic implications for basal Glires. J. Mamm. Evol. 8, 1–71. doi: 10.1023/A:1011328616715
Meng, J., Bowen, G. J., Ye, J., Koch, P. L., Ting, S. Y., Li, Q., et al. (2004). Gomphos elkema (Glires, Mammalia) from the erlian basin: evidence for the early tertiary bumbanian land mammal age in Nei-Mongol, China. Am. Mus. Nov. 3425, 1–24. doi: 10.1206/0003-00822004425<0001:GEGMFT<2.0.CO;2
Meng, J., Hu, Y., and Li, C. K. (2005). Gobiolagus (Lagomorpha, Mammalia) from Eocene Ula Usu, Inner Mongolia, and comments on Eocene lagomorphs of Asia. Palaeont. Electr. 8:23.
Meng, J., Hu, Y.-M., and Li, C.-K. (2003). The osteology of Rhombomylus (Mammalia, Glires): implications for phylogeny and evolution of Glires. Bull. Am. Mus. Nat. Hist. 275, 1–247. doi: 10.1206/0003-00902003275<0001:TOORMG<2.0.CO;2
Meng, J., Wyss, A. R., Dawson, M. R., and Zhai, R. (1994). Primitive fossil rodent from Inner Mongolia and its implications for mammalian phylogeny. Nature 370, 134–136. doi: 10.1038/370134a0
Metscher, B. D. (2009a). Micro-CT for comparative morphology: simple staining methods allow high-contrast 3D imaging of diverse non-mineralized animal tissues. BMC Physiol. 9:11. doi: 10.1186/1472-6793-9-11
Metscher, B. D. (2009b). Micro-CT for developmental biology: a versatile tool for high-contrast 3-D imaging at histological resolutions. Dev. Dyn. 238, 632–640. doi: 10.1002/dvdy.21857
Mohammadi, Z., Aliabadian, M., Ghorbani, F., Moghaddam, F. Y., Lissovsky, A. A., Obst, M., et al. (2020). Unidirectional introgression and evidence of hybrid superiority over parental populations in Eastern Iranian Plateau population of hares (Mammalia: Lepus Linnaeus, 1758). J. Mamm. Evol. 27, 723–743. doi: 10.1007/s10914-019-09478-5
Monaghan, P. (2014). Behavioral ecology and the successful integration of function and mechanism. Behav. Ecol. 25, 1019–1021. doi: 10.1093/beheco/aru082
Mooney, M. P., Siegel, M. I., Burrows, A. M., Smith, T. D., Losken, H. W., Dechant, J., et al. (1998a). A rabbit model of human familial, nonsyndromic unicoronal suture synostosis I. Synostotic onset, pathology, and sutural growth patterns. Child’s Nerv. Syst. 14, 236–246.
Mooney, M. P., Siegel, M. I., Burrows, A. M., Smith, T. D., Losken, H. W., Dechant, J., et al. (1998b). A rabbit model of human familial, nonsyndromic unicoronal suture synostosis II. Intracranial contents, intracranial volume, and intracranial pressure. Child’s Nerv. Syst. 14, 247–255. doi: 10.1007/s003810050220
Moss, M. L., and Feliciano, W. C. (1977). A functional analysis of the fenestrated maxillary bone of the rabbit (Oryctolagus cuniculus). Anat. Histol. Embryol. 6, 167–187. doi: 10.1111/j.1439-0264.1977.tb00431.x
Murphy, W. J., Eizirik, E., O’Brien, S. J., Madsen, O., Scally, M., Douady, C. J., et al. (2001). Resolution of the early placental mammal radiation using Bayesian phylogenetics. Science 294, 2348–2351. doi: 10.1126/science.1067179
Myers, P., Espinosa, R., Parr, C. S., Jones, T., Hammond, G. S., and Dewey, T. A. (2020). The Animal Diversity Web. Available online at: https://animaldiversity.org (accessed on 23 November 2020)
Naff, K. A., and Craig, S. (2012). “Chapter 6 - The domestic rabbit, Oryctolagus cuniculus: origins and history,” in The Laboratory Rabbit, Guinea Pig, Hamster, and Other Rodents. American College of Laboratory Animal Medicine, eds M. A. Suckow, K. A. Stevens, and R. P. Wilson (Cambridge, MA: Academic Press), 157–163.
Nett, E. M., Jaglowski, B. A., Ravosa, L. J., Ravosa, D. D., and Ravosa, M. J. (in press). Food mechanical properties and cyclical loading in the feeding apparatus of llamas. J Mammal.
Neves, F., Agueda-Pinto, A., Pinheiro, A., Abrantes, J., and Esteves, P. J. (2019). Strong selection of the TLR2 coding region among the Lagomorpha suggests an evolutionary history that differs from other mammals. Immunogenetics 71, 437–443. doi: 10.1007/s00251-019-01110-3
Neyt, J. G., Buckwalter, J. A., and Carroll, N. C. (1998). Use of animal models in musculoskeletal research. Iowa Orthop. J. 18, 118–123.
Niu, Y., Wie, F., Li, M., Liu, X., and Feng, Z. (2004). Phylogeny of pikas (Lagomorpha, Ochotona) inferred from mitochondrial cytochrome b sequences. Folia Zool. 53, 141–155.
Novacek, M. J. (1993). “Patterns of diversity in the mammalian skull,” in The Skull, Vol. II, eds J. Hanken and B. K. Hall (Chicago, IL: University Press), 438–545.
Okuda, A., Hori, Y., Ichihara, N., Asari, M., and Wiggs, R. B. (2007). Comparative observation of skeletal-dental abnormalities in wild, domestic, and laboratory rabbits. J. Vet. Dent. 24, 224–229. doi: 10.1177/089875640702400403
Olson, E. C. (1942). The skull of Megalagus turgidus (Cope). Am. J. Sci. 240, 505–511. doi: 10.2475/ajs.240.7.505
Orliac, M. J., Ladevèze, S., Gingerich, P. D., Lebrun, R., and Smith, T. (2014). Endocranial morphology of Palaeocene Plesiadapis tricuspidens and evolution of the early primate brain. Proc. R. Soc. B 281:20132792. doi: 10.1098/rspb.2013.2792
Orr, R. T. (1940). The rabbits of California. occasional papers of the California. Acad. Sci. 19, 1–227.
Parés-Casanova, P. M., and Cabello, M. (2020). Patterns of mandibular asymmetries in two types of companion rabbits. Anat. Histol. Embr. 49, 227–232. doi: 10.1111/ahe.12517
Patnaik, R. (2002). Pliocene Leporidae (Lagomorpha, Mammalia) from the Upper Siwaliks of India: implications for phylogenetic relationships. J. Vertebr. Paleontol. 22, 443–452. doi: 10.1671/0272-46342002022[0443:PLLMFT]2.0.CO;2
Pinheiro, A., de Sousa-Pereira, P., Almeida, T., Ferreira, C. C., Otis, J. A., Boudreau, M. R., et al. (2019). Sequencing of VDJ genes in Lepus americanus confirms a correlation between VHn expression and the leporid species continent of origin. Mol. Immunol. 112, 182–187. doi: 10.1016/j.molimm.2019.05.008
Pinheiro, A., de Sousa-Pereira, P., Strive, T., Knight, K. L., Woof, J. M., Esteves, P. J., et al. (2018). Identification of a new European rabbit IgA with a serine-rich hinge region. PLoS One 13:e0201567. doi: 10.1371/journal.pone.0201567
Pinheiro, A., Neves, F. Lemos, de Matos, A., Abrantes, J., van der Loo, W., et al. (2016). An overview of the lagomorph immune system and its genetic diversity. Immunogenetics 68, 83–107. doi: 10.1007/s00251-015-0868-8
Propper, A. Y. (1976). Modalités et déterminisme du développement embryonnaire de la glande mammaire. Senologia 1, 19–26.
Racicot, R. A. (2017). “Fossil secrets revealed: X-ray CT scanning and applications in paleontology. Paleontol. Soc. Pap. 22, 21–38.
Racicot, R., and Ruf, I. (2020). Data from: MicroCT Scans of an Iodine Stained Prenatal Rabbit. MorphoSource. Available online at: http://www.morphosource.org/Detail/ProjectDetail/Show/project_id/1184 (accessed November 25, 2020).
Ravosa, M. J., and Hogue, A. S. (2004). “Function and fusion of the mandibular symphysis in mammals: a comparative and experimental perspective,” in Anthropoid Evolution. New Visions, eds C. F. Ross and R. F. Kay (New York, NY: Springer/Kluwer Academic Publishers), 413–462.
Ravosa, M. J., and Kane, R. J. (2017). Dietary variation and mechanical properties of articular cartilage in the temporomandibular joint: implications for the role of plasticity in mechanobiology and pathobiology. Zoology 124, 42–50. doi: 10.1016/j.zool.2017.08.008
Ravosa, M. J., Kunwar, R., Stock, S., and Stack, M. S. (2007). Pushing the limit: masticatory stress and adaptive plasticity in mammalian craniomandibular joints. J. Exp. Biol. 210, 628–641. doi: 10.1242/jeb.02683
Ravosa, M. J., López, E. K., Menegaz, R. A., Stock, S. R., Stack, M. S., and Hamrick, M. W. (2008). “Adaptive plasticity in the mammalian masticatory complex: you are what, and how, you eat,” in Primate Craniofacial Biology and Function, eds C. J. Vinyard, M. J. Ravosa, and C. E. Wall (New York, NY: Springer), 293–328.
Ravosa, M. J., Menegaz, R. A., Scott, J. E., Daegling, D. J., and McAbee, K. R. (2016). Limitations of a morphological criterion of adaptive inference in the fossil record. Biol. Rev. 91, 883–898. doi: 10.1111/brv.12199
Ravosa, M. J., Ning, J., Costley, D. B., Daniel, A. N., Stock, S. R., and Stack, M. S. (2010a). Masticatory biomechanics and masseter fiber-type plasticity. J. Musculoskel. Neuron. 10, 46–55.
Ravosa, M. J., Ross, C. F., Williams, S. H., and Costley, D. B. (2010b). Allometry of masticatory loading parameters in mammals. Anat. Rec. 293, 557–571. doi: 10.1002/ar.21133
Ravosa, M. J., Scott, J. E., McAbee, K. R., Veit, A. J., and Fling, A. L. (2015). Chewed out: An experimental link between food mechanical properties and repetitive loading of the masticatory apparatus in mammals. PeerJ 3:e1345. doi: 10.7717/peerj.1345
Reese, A. T., Lanier, H. C., and Sargis, E. J. (2013). Skeletal indicators of ecological specialization in pika (Mammalia, Ochotonidae). J. Morphol. 274, 585–602. doi: 10.1002/jmor.20127
Robinson, T., and Matthee, C. (2005). Phylogeny and evolutionary origins of the Leporidae: a review of cytogenetics, molecular analyses and a supermatrix analysis. Mammal. Rev. 35, 231–247. doi: 10.1111/j.1365-2907.2005.00073.x
Rodriguez, J. M., Sanz, J., Alonso, F., and Acosta, M. (1985). Factores que influyen en la duracion de la gestacion de la coneja. Arch. Zootec. 34, 183–194.
Ruf, I. (2014). Comparative anatomy and systematic implications of the turbinal skeleton in Lagomorpha (Mammalia). Anat. Rec. 297, 2031–2046. doi: 10.1002/ar.23027
Ruf, I. (2020). Ontogenetic transformations of the ethmoidal region in Muroidea (Rodentia, Mammalia): new insights from perinatal stages. Vert. Zool. 70, 383–415. doi: 10.26049/VZ70-3-2020-10
Ruf, I., Frahnert, S., and Maier, W. (2009). The chorda tympani and its significance for rodent phylogeny. Mamm. Biol. 74, 100–113. doi: 10.1016/j.mambio.2008.01.002
Ruf, I., Meng, J., and Fostowicz-Frelik, Ł (2021). Anatomy of the nasal and auditory regions of the fossil lagomorph Palaeolagus haydeni: systematic and evolutionary implications. Front. Ecol. Evol. 9:636110. doi: 10.3389/fevo.2021.636110
Ruf, I., Schubert, A. M., and Koenigswald, W. V. (2020). “Case studies on functional aspects and constraints in early and late tooth ontogeny,” in Mammal Teeth – Form and Function, eds T. Martin and W. V. Koenigswald (München: Verlag Dr. Friedrich Pfeil), 102–124.
Sánchez-Villagra, M. R., and Forasiepi, A. M. (2017). On the development of the chondrocranium and the histological anatomy of the head in perinatal stages of marsupial mammals. Zool. Lett. 3:1. doi: 10.1186/s40851-017-0062-y
Sánchez-Villagra, M. R., Segura, V., Geiger, M., Heck, L., Veitschegger, K., and Flores, D. (2017). On the lack of a universal pattern associated with mammalian domestication: differences in skull growth trajectories across phylogeny. R. Soc. Open Sci. 4:170876. doi: 10.1098/rsos.170876
Sanger, T. J., and Kircher, B. K. (2017). “Model clades versus model species: Anolis lizards as an integrative model of anatomical evolution,” in Avian and Reptilian Developmental Biology: Methods and Protocols, ed. G. Sheng (New York, NY: Humana Press), 285–297.
Sanger, T. J., Revell, L. J., Gibson-Brown, J. J., and Losos, J. B. (2012). Repeated modification of early limb morphogenesis programmes underlies the convergence of relative limb length in Anolis lizards. Proc. R. Soc. B 279, 739–748. doi: 10.1098/rspb.2011.0840
Schai-Braun, S. C., and Hackländer, K. (2016). “Leporidae (hares and rabbits),” in Handbook of the Mammals of the World. Vol. 6. Lagomorphs and Rodents I, eds D. E. Wilson, T. E. Lacher, and R. A. Mittermeier (Barcelona: Lynx Edicions), 62–148.
Schilling, N., and Hackert, R. (2006). Sagittal spine movements of small therian mammals during asymmetrical gaits. J. Exp. Biol. 209, 3925–3939. doi: 10.1242/jeb.02400
Scott, J. E., McAbee, K. R., Eastman, M. M., and Ravosa, M. J. (2014a). Experimental perspective on fallback foods and dietary adaptations in early hominins. Biol. Lett. 10:20130789. doi: 10.1098/rsbl.2013.0789
Scott, J. E., McAbee, K. R., Eastman, M. M., and Ravosa, M. J. (2014b). Teaching an old jaw new tricks: diet-induced plasticity in a model organism from weaning to adulthood. J. Exp. Biol. 217, 4099–4107. doi: 10.1242/jeb.111708
Seixas, F. A., Boursot, P., and Melo-Ferreira, J. (2018). The genomic impact of historical hybridization with massive mitochondrial DNA introgression. Genome Biol. 19:91. doi: 10.1186/s13059-018-1471-8
Shan, W., Zhang, Y., Tursun, M., Zhou, S., Dai, H., and Maimaiti, M. (2020). The complete mitochondrial genome sequence of Lepus tibetanus pamirensis. Mitochondr. DNA Part B 5, 1359–1360. doi: 10.1080/23802359.2020.1736959
Sherratt, E., del Rosario, Castañeda, M., Garwood, R. J., Mahler, D. L., Sanger, T. J., et al. (2015). Amber fossils demonstrate deep-time stability of Caribbean lizard communities. PNAS 112, 9961–9966. doi: 10.1073/pnas.1506516112
Silcox, M. T., Benham, A. E., and Bloch, J. I. (2010). Endocasts of Microsyops (Microsyopidae, Primates) and the evolution of the brain in primitive primates. J. Hum. Evol. 58, 505–521. doi: 10.1016/j.jhevol.2010.03.008
Silcox, M. T., Dalmyn, C. K., and Bloch, J. I. (2009). Virtual endocast of Ignacius graybullianus (Paromomyidae, Primates) and brain evolution in early Primates. PNAS 106, 10987–10992. doi: 10.1073/pnas.0812140106
Simons, R. S. (1999). Running, breathing and visceral motion in the domestic rabbit (Oryctolagus cuniculus): testing visceral displacement hypotheses. J. Exp. Biol. 202, 563–577.
Smith, A. T., Johnston, C. H., Alves, P. C., and Hackländer, K. (2018). Lagomorphs: Pikas, Rabbits, and Hares of the World. Baltimore: Johns Hopkins University Press.
Stanhope, M. J., Czelusniak, J., Si, J. S., Nickerson, J., and Goodman, M. (1992). A molecular perspective on mammalian evolution from the gene encoding interphotoreceptor retinoid binding protein, with convincing evidence for bat monophyly. Mol. Phylogenet. Evol. 1, 148–160. doi: 10.1016/1055-7903(92)90026-D
Stanhope, M. J., Smith, M. R., Waddell, V. G., Porter, C. A., Shivji, M. S., and Goodman, M. (1996). Mammalian evolution and the interphotoreceptor retinoid binding protein (IRBP) gene: convincing evidence for several superordinal clades. J. Mol. Evol. 43, 83–92.
Stock, A. D. (1976). Chromosome banding pattern relationships of hares, rabbits, and pikas (order Lagomorpha). A phyletic interpretation. Cytogenet. Cell. Genet. 17, 78–88. doi: 10.1159/000130692
Stoner, C. J., Bininda-Emonds, O. R. P., and Caro, T. (2003). The adaptive significance of coloration in lagomorphs. Biol. J. Linn. Soc. 79, 309–328. doi: 10.1046/j.1095-8312.2003.00190.x
Stott, P., Jennings, N., and Harris, S. (2010). Is the large size of the pinna of the ear of the European hare (Lepus europaeus) due to its role in thermoregulation or in anterior capital shock absorption? J. Morphol. 271, 674–681. doi: 10.1002/jmor.10825
Stübinger, S., and Dard, M. (2013). The rabbit as experimental model for research in implant dentistry and related tissue regeneration. J. Investig. Surg. 26, 266–282. doi: 10.3109/08941939.2013.778922
Sych, L. (1967). Fossil endocranial cast of Hypolagus brachygnathus Kormos (Leporidae, Mammalia). Acta Zool. Crac. 12, 27–30.
Terhune, C. E., Sylvester, A. D., Scott, J. E., and Ravosa, M. J. (2020). Trabecular architecture of the mandibular condyle of rabbits is related to dietary resistance during growth. J Exp. Biol. 223:jeb220988s. doi: 10.1242/jeb.220988
Thibault, C. (1967). Analyse comparée de la fécondation et de ses anomalies chez la brebis, la vache et la lapine. Ann. Biol. Anim. Bioch. Biophys. 7, 5–23.
Tobien, H. (1974). Zur Gebißstruktur, Systematik und Evolution der Genera Amphilagus und Titanomys (Lagomorpha, Mammalia) aus einigen Vorkommen im jüngeren Tertiär Mittel-und Westeuropas. Mainz. Geowiss. Mitt. 3, 95–214.
Tobien, H. (1975). Zur Gebißstruktur, Systematik und Evolution der Genera Piezodus, Prolagus und Ptychoprolagus (Lagomorpha, Mammalia) aus einigen Vorkommen im jüngeren Tertiär Mittel- und Westeuropas. Notizbl. Hess. Ldsamt. Bodenforsch. Wiesb. 103, 103–186.
Tong, Y. (1997). Middle Eocene small mammals from Liguanqiao Basin of Henan Province and Yuanqu Basin of Shanxi Province, Central China. Palaeont. Sin. C 26, 1–256.
Upham, N. S., Esselstyn, J. A., and Jetz, W. (2019). Inferring the mammal tree: species-level sets of phylogenies for questions in ecology, evolution, and conservation. PLoS Biol. 17:e3000494. doi: 10.1371/journal.pbio.3000494
Velasco, J. A., Villalobos, F., Diniz–Filho, J. A. F., Poe, S., and Flores–Villela, O. (2020). Macroecology and macroevolution of body size in Anolis lizards. Ecography 43, 812–822. doi: 10.1111/ecog.04583
Vianey-Liaud, M., and Lebrun, R. (2013). New data about the oldest European lagomorpha: description of the new genus Ephemerolagus nievae gen. nov. et sp. nov. Span. J. Palaeont. 28, 3–16.
Vinyard, C. J., Ravosa, M. J., Williams, S. H., Wall, C. E., Johnson, K. R., and Hylander, W. L. (2006). “Jaw muscle function and the origin of primates,” in Primate Origins: Adaptations and Evolution, eds M. J. Ravosa and M. Dagosto (New York, NY: Springer), 179–231.
Vinyard, C. J., Yamashita, N., and Tan, C. L. (2008). Linking laboratory and field approaches in studying the evolutionary physiology of biting in bamboo lemurs. Int. J. Primatol. 29, 1421–1439. doi: 10.1007/s10764-007-9178-9
Voit, M. (1909). Das Primordialcranium des Kaninchens unter Berücksichtigung der Deckknochen. Anat. Hefte 38, 425–616.
Waltari, E., and Cook, J. A. (2005). Hares on ice: phylogeography and historical demographics of Lepus arcticus, L. othus, and L. timidus (Mammalia: Lagomorpha). Mol. Ecol. 14, 3005–3016. doi: 10.1111/j.1365-294X.2005.02625.x
Wang, X., Liang, D., Jin, W., Tang, M., and Shalayiwu, and Liu, S. (2020). Out of Tibet: genomic perspectives on the evolutionary history of extant pikas. Mol. Biol. Evol. 37, 1577–1592. doi: 10.1093/molbev/msaa026
Wang, X.-M., Qiu, Z.-D., Li, Q., Tomida, Y., Kimura, Y., Tseng, Z. J., et al. (2009). A new Early to Late Miocene fossiliferous region in central Nei Mongol: lithostratigraphy and biostratigraphy in Aoerban strata. Vertebr. PalAsiatica 47, 111–134.
Wang, Y. Q., Meng, J., Beard, K. C., Li, Q., Ni, X. J., Gebo, D. L., et al. (2010). Early Paleogene stratigraphic sequences, mammalian evolution and its response to environmental changes in Erlian Basin, Inner Mongolia, China. Sci. China Earth Sci. 53, 1918–1926. doi: 10.1007/s11430-010-4095-8
Watson, P. J., Gröning, F., Curtis, N., Fitton, L. C., Herrel, A., McCormack, S. W., et al. (2014). Masticatory biomechanics in the rabbit: a multi-body dynamics analysis. J. R. Soc. Interf. 11:20140564. doi: 10.1098/rsif.2014.0564
Weijs, W. A. (1980). Biomechanical models and the analysis of form: a study of the mammalian masticatory apparatus. Am. Zool. 20, 707–719. doi: 10.1093/icb/20.4.707
Weijs, W. A., and Dantuma, R. (1981). Functional anatomy of the masticatory apparatus in the rabbit (Oryctolagus cuniculus L.). Neth. J. Zool. 31, 99–147. doi: 10.1163/002829680X00212
Weijs, W. A., and de Jongh, H. J. (1977). Strain in mandibular alveolar bone during mastication in the rabbit. Arch. Oral Biol. 22, 667–675. doi: 10.1016/0003-9969(77)90096-6
Weijs, W. A., Brugman, P., and Grimbergen, C. A. (1989). Jaw movements and muscle activity during mastication in growing rabbits. Anat. Rec. 224, 407–416. doi: 10.1002/ar.1092240309
Weijs, W. A., Brugman, P., and Klok, E. M. (1987). The growth of the skull and jaw muscles and its functional consequences in the New Zealand rabbit Oryctolagus cuniculus. J. Morphol. 194, 143–161. doi: 10.1002/jmor.1051940204
White, J. A. (1991). North American Leporinae (Mammalia: Lagomorpha) from late Miocene (Clarendonian) to latest Pliocene (Blancan). J. Vertebr. Paleontol. 11, 67–89. doi: 10.1080/02724634.1991.10011376
Wible, J. R. (2007). On the cranial osteology of the Lagomorpha. Bull. Carnegie Mus. Nat. Hist. 39, 213–234. doi: 10.2992/0145-9058200739[213:OTCOOT]2.0.CO;2
Williams, S. B., Payne, R. C., and Wilson, A. M. (2007a). Functional specialisation of the pelvic limb of the hare (Lepus europeus). J. Anat. 210, 472–490. doi: 10.1111/j.1469-7580.2007.00704.x
Williams, S. B., Wilson, A. M., and Payne, R. C. (2007b). Functional specialisation of the thoracic limb of the hare (Lepus europeus). J. Anat. 210, 491–505. doi: 10.1111/j.1469-7580.2007.00703.x
Winkler, A. J., and Avery, D. M. (2010). “Lagomorpha,” in Cenozoic Mammals of Africa, eds L. Werdelin and W. J. Sanders (New York, NY: University of California Press), 169–317.
Winkler, A. J., and Tomida, Y. (2011). “Chapter 3 The lower third premolar of Serengetilagus praecapensis (Mammalia: Lagomorpha: Leporidae) from Laetoli, Tanzania,” in Paleontology and Geology of Laetoli: Human Evolution in Context. Volume 2: Fossil Hominins and the Associated Fauna, Vertebrate Paleobiology and Paleoanthropology, ed. T. Harrison (Berlin: Springer Science+Business Media B.V.).
Winkler, A. J., Winkler, D. A., and Harrison, T. (2016). Forelimb anatomy of Serengetilagus praecapensis (Mammalia: Lagomorpha): a Pliocene leporid from Laetoli, Tanzania. Hist. Biol. 28, 252–263. doi: 10.1080/08912963.2015.1023302
Witte, H., Biltzinger, J., Hackert, R., Schilling, N., Schmidt, M., Reich, C., et al. (2002). Torque patterns of the limbs of small therian mammals during locomotion on flat ground. J. Exp. Biol. 205, 1339–1353.
Wood, A. E. (1940). The mammalian fauna of the White River Oligocene. Part III. Lagomorpha. Trans. Am. Phil. Soc. 28, 271–362. doi: 10.2307/1005524
Wu, C., Wu, J., Bunch, T. D., Li, Q., Wang, Y., and Zhang, Y. P. (2005). Molecular phylogenetics and biogeography of Lepus in Eastern Asia based on mitochondrial DNA sequences. Mol. Phylogenet. Evol. 37, 45–61. doi: 10.1016/j.ympev.2005.05.006
Wu, Y., Xia, L., Zhang, Q., Yang, Q., and Meng, X. (2011). Bidirectional introgressive hybridization between Lepus capensis and Lepus yarkandensis. Mol. Phylogenet. Evol. 59, 545–555. doi: 10.1016/j.ympev.2011.03.027
Yamada, K., and Kimmel, D. B. (1991). The effect of dietary consistency on bone mass and turnover in the growing rat mandible. Arch. Oral Biol. 36, 129–138. doi: 10.1016/0003-9969(91)90075-6
Young, J. W., Danczak, R., Russo, G. A., and Fellmann, C. D. (2014). Limb bone morphology, bone strength, and cursoriality in lagomorphs. J. Anat. 225, 403–418. doi: 10.1111/joa.12220
Yu, N., Zheng, C., Shi, L., Wang, W., Lan, H., and Zhang, Y. (1996). Phylogenetic relationships of five pika species (genus Ochotona) based on mitochondrial DNA restriction maps. Sci. China C Life Sci. 39, 113–122. doi: 10.1360/yc1996-39-2-113
Yu, N., Zheng, C. L., Zhang, Y. P., and Li, W. H. (2000). Molecular systematics of pikas (genus Ochotona) inferred from mitochondrial DNA sequences. Mol. Phylogenet. Evol. 16, 85–95. doi: 10.1006/mpev.2000.0776
Zhang, Z. Q., and Wang, J. (2016). On the geological age of mammalian fossils from Shanmacheng, Gansu Province. Vertebr. PalAsiatica 54, 351–357.
Keywords: Lagomorpha, Leporidae, Ochotonidae, evolution, morphofunction, model organism, morphology, phylogeny
Citation: Kraatz B, Belabbas R, Fostowicz-Frelik Ł, Ge D-Y, Kuznetsov AN, Lang MM, López-Torres S, Mohammadi Z, Racicot RA, Ravosa MJ, Sharp AC, Sherratt E, Silcox MT, Słowiak J, Winkler AJ and Ruf I (2021) Lagomorpha as a Model Morphological System. Front. Ecol. Evol. 9:636402. doi: 10.3389/fevo.2021.636402
Received: 04 January 2021; Accepted: 14 May 2021;
Published: 01 July 2021.
Edited by:
Rodney L. Honeycutt, Pepperdine University, United StatesReviewed by:
Conrad Matthee, Stellenbosch University, South AfricaJohn Wible, Carnegie Museum of Natural History, United States
Thomas Martin, University of Bonn, Germany
Copyright © 2021 Kraatz, Belabbas, Fostowicz-Frelik, Ge, Kuznetsov, Lang, López-Torres, Mohammadi, Racicot, Ravosa, Sharp, Sherratt, Silcox, Słowiak, Winkler and Ruf. This is an open-access article distributed under the terms of the Creative Commons Attribution License (CC BY). The use, distribution or reproduction in other forums is permitted, provided the original author(s) and the copyright owner(s) are credited and that the original publication in this journal is cited, in accordance with accepted academic practice. No use, distribution or reproduction is permitted which does not comply with these terms.
*Correspondence: Brian Kraatz, bkraatz@westernu.edu