From Sanford Underground Research Facility
February 4, 2019
Erin Broberg
Researchers recently got a special delivery: a hundred million atoms of Cobalt-56, an ideal calibration source.
A string of germanium detectors inside a cleanroom glovebox on the 4850 Level of Sanford Lab, before they were installed in the Majorana Demonstrator in 2016.
Photo by Matthew Kapust

Researchers have not seen the copper glow of the Majorana Demonstrator’s internal detector since 2016. Sealed behind six layers, including 5,200 lead bricks and two heavy copper shields, the Majorana Demonstrator has recorded a steady stream of data that will inform the next-generation neutrinoless double-beta decay experiments. But how do researchers know if the information they’re receiving is accurate? How do they know something hasn’t gone amiss deep inside?
Simple. They use an advanced calibration system that allows them to monitor the performance of the germanium detectors that make up the heart of the demonstrator. Ralph Massarczyk, staff scientist at Los Alamos National Laboratory, designed and created the calibration system used by the Majorana Demonstrator collaboration.
“In a typical detector,” Massarczyk explains, “there is enough natural background that you can easily calibrate a detector. But with Majorana, you have a very minimal background, which is not enough to determine its performance.”
Without substantial background data, researchers don’t know if the background is stable or not. The detector could be reporting events at inaccurate energy levels or even missing them completely. So, to calibrate this extremely sensitive detector, a calibration source is used to produce a standard set of well-known physics events that researchers can use to understand detector performance.
Typically, the collaboration uses thorium, a naturally occurring, slightly radioactive material that creates signatures the Majorana Demonstrator can easily read. The only problem with this source is that the signatures it produces are at a slightly higher energy level than that at which neutrinoless double-beta decay is expected to occur.
For a more ideal calibration, Massarczyk and his team got a special delivery: a hundred million atoms of Cobalt-56, a slightly radioactive isotope created in particle accelerators and used mostly in the medical field. The source underwent several “swipe tests” to ensure no leaks had occurred.
“Cobalt-56 is an ideal source. It produces a lot of events, and those events are at the exact energy where we expect to see a neutrinoless double-beta decay event,” Massarczyk said.
If it is such a perfect indicator, why don’t researchers use it every time?
“Cobalt-56 has a really short half-life, only 77 days,” said Massarczyk. “This means that at the end of 77 days, only one-half of the source will be left. After waiting another 77 days, only one-fourth will be left. After a year, the source is gone.”
Thorium, on the other hand, lasts for years. Indeed, the collaboration has been using its thorium source for five years, Massarczyk said.
Delivery methods
To deliver a calibration source to the detector modules behind layers of shielding, Massarczyk designed a “line source.” In this system, a 5-meter long, half-inch thick plastic tube is inserted into a track from the outside of the shield. The tube, which carries the calibration source, is pushed along the “grooves” on the outside of each detector module, snaking its way around twice.
“It sort of resembles a helix,” Massarczyk said. “This way, the signals are distributed evenly, rather than coming from one point, allowing each detector within the modules to see activity from the same source.”
The normal rate for the Majorana Demonstrator is a few signature counts per hour. When a radioactive calibration source is included, researchers see a few thousand events per second. During its weekly calibration run, the Majorana Demonstrator sees more events in 3 hours than it would otherwise detect in the span of 120 years.
“If, while this source is inside, the demonstrator creates signals that correspond with known data, then we know the demonstrator is well-calibrated and on track,” Massarczyk said.
Looking to the future
The Majorana Demonstrator is expected to run for a few more years, so the short half-life of Cobalt-56 means it is not a sustainable calibration option for the team. That’s why this week’s calibration was so important. The data collected has been sent to analysts for interpretation.
“The main purpose for this data is to double-check the data analysis we do in the energy region 2MeV, where we expect the neutrinoless double-beta decay events to occur,” Massarczyk said.
The information gained from these tests also is of interest to collaborators with LEGEND (Large Enriched Germanium Experiment for Neutrinoless ββ Decay), who are trying to perfect the next-generation neutrinoless double-beta decay experiment.
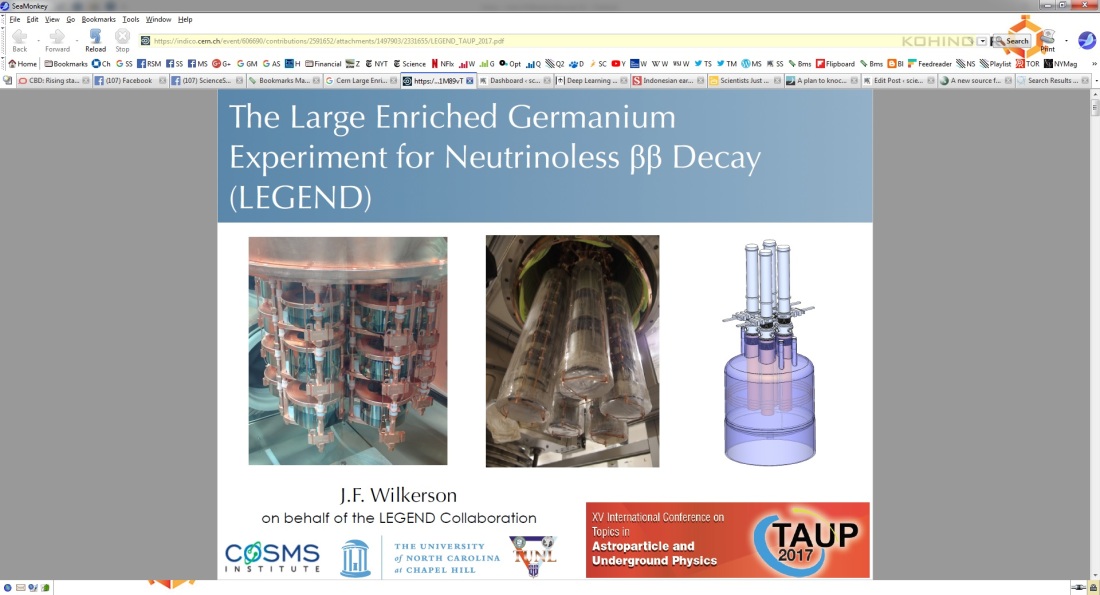
“As they plan a ton-scale experiment, researchers want to know if the materials are clean enough, if the shielding is working and how far underground they need to go,” said Massarczyk. “Understanding the backgrounds gives us important information to make those decisions.”
See the full article here .
five-ways-keep-your-child-safe-school-shootings
Please help promote STEM in your local schools.
About us.
The Sanford Underground Research Facility in Lead, South Dakota, advances our understanding of the universe by providing laboratory space deep underground, where sensitive physics experiments can be shielded from cosmic radiation. Researchers at the Sanford Lab explore some of the most challenging questions facing 21st century physics, such as the origin of matter, the nature of dark matter and the properties of neutrinos. The facility also hosts experiments in other disciplines—including geology, biology and engineering.
The Sanford Lab is located at the former Homestake gold mine, which was a physics landmark long before being converted into a dedicated science facility. Nuclear chemist Ray Davis earned a share of the Nobel Prize for Physics in 2002 for a solar neutrino experiment he installed 4,850 feet underground in the mine.
Homestake closed in 2003, but the company donated the property to South Dakota in 2006 for use as an underground laboratory. That same year, philanthropist T. Denny Sanford donated $70 million to the project. The South Dakota Legislature also created the South Dakota Science and Technology Authority to operate the lab. The state Legislature has committed more than $40 million in state funds to the project, and South Dakota also obtained a $10 million Community Development Block Grant to help rehabilitate the facility.
In 2007, after the National Science Foundation named Homestake as the preferred site for a proposed national Deep Underground Science and Engineering Laboratory (DUSEL), the South Dakota Science and Technology Authority (SDSTA) began reopening the former gold mine.
In December 2010, the National Science Board decided not to fund further design of DUSEL. However, in 2011 the Department of Energy, through the Lawrence Berkeley National Laboratory, agreed to support ongoing science operations at Sanford Lab, while investigating how to use the underground research facility for other longer-term experiments. The SDSTA, which owns Sanford Lab, continues to operate the facility under that agreement with Berkeley Lab.
The first two major physics experiments at the Sanford Lab are 4,850 feet underground in an area called the Davis Campus, named for the late Ray Davis. The Large Underground Xenon (LUX) experiment is housed in the same cavern excavated for Ray Davis’s experiment in the 1960s.
LUX/Dark matter experiment at SURF
In October 2013, after an initial run of 80 days, LUX was determined to be the most sensitive detector yet to search for dark matter—a mysterious, yet-to-be-detected substance thought to be the most prevalent matter in the universe. The Majorana Demonstrator experiment, also on the 4850 Level, is searching for a rare phenomenon called “neutrinoless double-beta decay” that could reveal whether subatomic particles called neutrinos can be their own antiparticle. Detection of neutrinoless double-beta decay could help determine why matter prevailed over antimatter. The Majorana Demonstrator experiment is adjacent to the original Davis cavern.
LUX’s mission was to scour the universe for WIMPs, vetoing all other signatures. It would continue to do just that for another three years before it was decommissioned in 2016.
In the midst of the excitement over first results, the LUX collaboration was already casting its gaze forward. Planning for a next-generation dark matter experiment at Sanford Lab was already under way. Named LUX-ZEPLIN (LZ), the next-generation experiment would increase the sensitivity of LUX 100 times.
SLAC physicist Tom Shutt, a previous co-spokesperson for LUX, said one goal of the experiment was to figure out how to build an even larger detector.
“LZ will be a thousand times more sensitive than the LUX detector,” Shutt said. “It will just begin to see an irreducible background of neutrinos that may ultimately set the limit to our ability to measure dark matter.”
We celebrate five years of LUX, and look into the steps being taken toward the much larger and far more sensitive experiment.
Another major experiment, the Long Baseline Neutrino Experiment (LBNE)—a collaboration with Fermi National Accelerator Laboratory (Fermilab) and Sanford Lab, is in the preliminary design stages. The project got a major boost last year when Congress approved and the president signed an Omnibus Appropriations bill that will fund LBNE operations through FY 2014. Called the “next frontier of particle physics,” LBNE will follow neutrinos as they travel 800 miles through the earth, from FermiLab in Batavia, Ill., to Sanford Lab.

The MAJORANA DEMONSTRATOR will contain 40 kg of germanium; up to 30 kg will be enriched to 86% in 76Ge. The DEMONSTRATOR will be deployed deep underground in an ultra-low-background shielded environment in the Sanford Underground Research Facility (SURF) in Lead, SD. The goal of the DEMONSTRATOR is to determine whether a future 1-tonne experiment can achieve a background goal of one count per tonne-year in a 4-keV region of interest around the 76Ge 0νββ Q-value at 2039 keV. MAJORANA plans to collaborate with GERDA for a future tonne-scale 76Ge 0νββ search.

CASPAR is a low-energy particle accelerator that allows researchers to study processes that take place inside collapsing stars.
The scientists are using space in the Sanford Underground Research Facility (SURF) in Lead, South Dakota, to work on a project called the Compact Accelerator System for Performing Astrophysical Research (CASPAR). CASPAR uses a low-energy particle accelerator that will allow researchers to mimic nuclear fusion reactions in stars. If successful, their findings could help complete our picture of how the elements in our universe are built. “Nuclear astrophysics is about what goes on inside the star, not outside of it,” said Dan Robertson, a Notre Dame assistant research professor of astrophysics working on CASPAR. “It is not observational, but experimental. The idea is to reproduce the stellar environment, to reproduce the reactions within a star.”